KEY POINTS
No hemodynamic monitoring device will improve patient outcome unless coupled to a treatment, which itself improves outcome.
Low venous oxygen saturations need not mean circulatory shock but do imply circulatory stress, as they may occur in the setting of hypoxemia, anemia, exercise, as well as circulatory shock.
There is no “normal” cardiac output, only one that is adequate or inadequate to meet the metabolic demands of the body. Thus, targeting a specific cardiac output value without reference to metabolic need, or oxygen-carrying capacity of the blood, is dangerous.
Cardiac output is estimated, not measured, by all devices routinely used in bedside monitoring (though we shall call it measured in this text).
Cardiac output estimates using arterial pulse pressure contour analysis cannot be interchanged among devices and all suffer to a greater or lesser extent by changes of peripheral vasomotor tone commonly seen in the critically ill.
Since metabolic demands can vary rapidly, continuous or frequent measures of cardiac output are preferred to single or widely spaced individual measures.
Integrating several physiologic variables in the assessment of the adequacy of the circulation usually gives a clearer picture than just looking at one variable.
Integrating cardiac output with other measures, like venous oxygen saturation, can be very helpful in defining the adequacy of blood flow.
INTRODUCTION
The goal of the cardiorespiratory system is to sustain adequate delivery of oxygen to the tissues and removal of carbon dioxide to meet their metabolic demands. Under normal conditions, this system has significant flow and O2-carrying capacity reserve to handle all but the most demanding metabolic stresses or primary organ dysfunction. Indeed, once overt cardiorespiratory failure is present, the degree of cardiorespiratory impairment is often advanced with existing end-organ ischemic dysfunction. Hemodynamic monitoring plays an important role in the management of these critically ill patients with cardiovascular dysfunction. It is profoundly useful in the titration of therapies in patients with known cardiovascular disease processes, like hemorrhagic shock, acute mitral regurgitation, cor pulmonale, left-sided heart failure, and vasoplegic shock wherein knowing the underlying pathophysiologic process, physiologic state, and titrating-specific therapies represents the centerpiece of cardiovascular support. However, monitoring is also useful in identifying problems before they deteriorate to shock and/or in the management of high-risk patients with proven therapies. In this chapter, we will discuss monitoring the elements of oxygen delivery (DO2), namely cardiac output and blood oxygen saturation. Hemoglobin is the other variable defining DO2, but usually does not change rapidly and can be estimated from venous oximetry. Although circulatory shock is due to inadequate DO2 to meet metabolic demands, targeting-specific cardiac output or DO2 values across all patients in an attempt to prevent occult or obvious tissue hypoperfusion and ischemia is not only unwarranted but potentially harmful, as the needs of different patients and the same patient over time can vary widely.
Recent reviews on the usefulness of hemodynamic monitoring to identify and monitor therapy have been written.1,2 Importantly, a recent consensus statement by 16 experts underscores many of the principles described below.3 The essential aspects of circulatory sufficiency, namely the adequacy of DO2 to sustain metabolic needs, can be assessed by a combination of routinely available variables. For convenience these will be separated into those that measure oxygenation (oximetry) and those that measure flow (cardiac output).
TISSUE OXYGENATION
Although mean arterial pressure is a primary determinant of organ perfusion, normotension can coexist with circulatory shock.
Since metabolic demand of tissues varies by external (exercise) and internal (basal metabolism, digestion, fever) stresses, there is no “normal” cardiac output that the bedside caregiver can target and be assured of perfusion adequacy. Cardiac output is either adequate or inadequate to meet the metabolic demands of the body. Thus, although measures of cardiac output are important, their absolute values are relevant only in the extremes and when targeting specific clinical conditions, such as preoptimization therapy, which will be described below. How then does one know that circulatory sufficiency is present or that circulatory shock exists? Clearly, since arterial pressure is the primary determinant of organ blood flow, systemic hypotension (ie, mean arterial pressure <60 mm Hg) must result in tissue hypotension.4 Organ perfusion pressure can be approximated as mean arterial pressure (MAP) relative to tissue or outflow pressure. If intracranial pressure or intra-abdominal pressure increases, then estimating cerebral or splanchnic perfusion pressure using MAP alone will grossly overestimate organ perfusion pressure. However, baroreceptors in the carotid body and aortic arch increase vasomotor tone to keep cerebral perfusion constant if flow decreases, and the associated increased systemic sympathetic tone alters local vasomotor tone to redistribute blood flow away from more efficient O2 extracting tissues to sustain MAP and global O2 consumption (˙VO2) in the setting of inappropriately decreasing DO2. Thus, although systemic hypotension is a medical emergency and reflects severe circulatory shock, the absence of systemic hypotension does not ensure that all tissues are being adequately perfused.
Within this context measures of tissue and blood oxygenation become relevant because they define the amount of O2 within the tissues or blood and their measures can often be made noninvasively and using indwelling venous or central venous catheters. This oximetric monitoring offers the potential to assess continuous measures of the adequacy of blood flow. Furthermore, measures of anaerobic metabolism, such as serum lactate levels or arterial blood gas base deficit, often confirm the existence of tissue ischemia.5,6 Noninvasive pulse oximetry allows for the continuous measure of arterial oxygen saturation and is universally used in acute care settings, despite no evidence that it improves outcomes.7 Pulmonary artery catheterization allows for the continuous measure of mixed venous oxygen saturation (SvO2) through reflectance oximetry. Central venous catheterization, commonly used to secure a stable intravascular infusion site, allows for the sampling of superior vena caval central venous oxygen saturation (ScvO2) and by reflectance oximetry continuous ScvO2)measures as well. The principles behind these measures, their usefulness, and limitations are described below.
Hemoglobin varies in its light absorption spectrum as it binds to oxygen, carbon monoxide, nothing (deoxygenation), or is in the ferric state (methemoglobin) (Fig. 32-1). All oximeters estimate blood or tissue O2 saturation by measuring the differential absorbance bands of various paired or triple spectral signals across the 600 to 1000 nm bandwidth.
Arterial blood O2 saturation (SaO2) can be estimated quite accurately at the bedside using pulse oximetry.8 The routine use of pulse oximetry has markedly reduced the number of arterial blood gases needed to manage patients with hypoxemic respiratory failure. Importantly, pulse oximeters estimate SaO2 saturation by measuring the change in tissue light absorption at two specific wavelengths, 660 nm (red) and 940 nm (infrared). In the steady state, the absorption ratios, based on calibration against known SaO2 values, allows for the continuous measure of total tissue absorption, which is a function of both arterial blood and all the other tissues and blood that are within the sensing oximeter’s path. However, the dynamic change in absorption induced by the arterial systolic pulse primarily increases the arterial and arteriolar blood volume. The pulse oximeter examines the change in absorption ratios of the pulse-induced change from the associated plethysmographic waveform. Since the SaO2 is sensed as pulsed O2 saturation, it is referred to as pulse O2 saturation (SpO2). Routinely, pulse oximeters are placed on a finger for convenience sake. However, if no pulse is sensed, then the readings are meaningless. Such finger pulselessness can be seen with peripheral vasoconstriction associated with hypothermia, circulatory shock, or vasospasm. Central pulse oximetry using transmission technology can be applied to the ear or bridge of the nose and reflectance oximetry can be applied to the forehead, all of which tend to retain pulsatility if central pulsatile flow is present. Similarly, during cardiopulmonary bypass when arterial flow is constant, pulse oximetry is inaccurate.
Clinical Uses of SpO2: The primary uses of SpO2 are for identification of arterial hypoxemia, titration of supplemental O2, and indirectly, to assess the causes of hypoxemia. An additional use of the plethysmographic waveform independent of measuring SpO2 is as a surrogate for arterial pulse pressure.
Hypoxemia: A primary cause of inadequate DO2 is arterial hypoxemia. SpO2 is routinely used to identify hypoxemia.9 Owing to the shape of the oxyhemoglobin dissociation curve, a PaO2 of 60 mm Hg represents an SpO2 of 90% (Fig. 32-2). Hypoxemia is usually defined as an SpO2 <90%. Increasing supplemental inspired oxygen (increased FiO2), positive end-expiratory pressure, and other ventilatory maneuvers can be titrated to keep SpO2 >90% using various respiratory treatment algorithms.
Identifying the causes of hypoxemia: The most common causes of hypoxemia are ventilation-perfusion (V/Q) mismatch and shunt. With V/Q mismatch alveolar hypoxia occurs in lung regions with increased flow relative to ventilation, such that the high blood flow rapidly depletes alveolar O2 before the next breath can refresh it. Accordingly, this process readily lends itself to improved oxygenation by increasing FiO2 and minimizing regional alveolar hypoxia. Collapsed or flooded lung units will not alter their alveolar O2 levels by this maneuver and are said to be refractory to increases in FiO2. Accordingly, by measuring the SpO2 response to slight increases in FiO2 one can separate V/Q mismatch from shunt. One merely measures SpO2 while switching from room air FiO2 of 0.21) to 2 to 4 L/min nasal cannula (FiO2 ∼0.3). Shunt can be due to anatomical intracardiac shunts, alveolar flooding (eg, pneumonia and pulmonary edema), and atelectasis. Importantly, atelectatic lung units should be recruitable by lung expansion whereas flooded lung units and anatomical shunts should not. Thus, by performing sustained deep inspirations and having the patient sit up and take deep breaths one should be able to separate easily recruitable atelectasis from true shunt. Sitting up and taking deep breaths is a form of exercise that may increase O2 extraction by the tissues, thus decreasing SvO2. The patient with atelectasis will increase alveolar ventilation increasing SpO2 despite the decrease in SvO2, whereas the patient with shunt or poorly recruitable atelectasis will realize a fall in SpO2 as the shunted blood will carry the lower SvO2 to the arterial side.
Detection of volume responsiveness: Recent interest in the clinical applications of heart-lung interactions has centered on the effect of positive-pressure ventilation on venous return and subsequently cardiac output. In those subjects who are volume responsive, arterial pulse pressure, as a measure of left ventricular (LV) stroke volume, phasically decreases in phase with expiration, the magnitude of which is proportional to their volume responsiveness.10,11 Since the pulse oximeter’s plethysmographic waveform is a manifestation of the arterial pulse pressure, if pulse pressure varies from beat-to-beat so will the plethysmographic deflection, which can be quantified. Several groups have documented that the maximal variations in pulse oximeter’s plethysmographic waveform during positive-pressure ventilation covaries with arterial pulse pressure variation and can be used in a similar fashion to identify those subjects who are volume responsive.12,13
Venous oximetry measures O2 saturation in venous blood, defining that residual O2 in that bed after traversing the tissue. The higher the venous O2 content, the more O2 remained following its transit through the tissues and presumably the greater the O2 extraction reserve. To the extent that SpO2 and hemoglobin concentration result in an adequate arterial O2 content (CaO2), then ScvO2 and SvO2 levels can be taken to reflect the adequacy of the circulation to meet the metabolic demands of the tissues. However, these assumptions are not always correct so that blind use of SvO2 or ScvO2 to assess circulatory sufficiency may lead to misdiagnosis. Thus, one needs to examine the determinants of DO2, global oxygen consumption (VO2), and effective O2 extraction by the tissues before using ScvO2 or SvO2 as markers of circulatory sufficiency. DO2 is the product of cardiac output and CaO2. CaO2 is the sum of oxygen bound to hemoglobin (product of hemoglobin concentration [Hb] and SaO2) and dissolved oxygen (PaO2). Although the formula defining CaO2 is Hb·1.36·SaO2 + PaO2·0.0031, the amount of O2 dissolved in the plasma is minimal except under extreme hyperbaric conditions, and is usually ignored.
Clinical Uses of SvO2: Since VO2 must equal cardiac output times the difference in CaO2 and mixed venous O2 content (CvO2), if CaO2 remains relatively constant then CvO2 will vary in proportion to cardiac output. Recall from above that SpO2 can be easily measured and Hb rarely changes rapidly, thus in the sedated patient without changing stress, this assumption is true. Since the amount of O2 dissolved in the plasma is very small, the primary factor determining changes in CvO2 will be SvO2. Thus, SvO2 correlates well with the O2 supply-to-demand ratio.14
However, several relevant conditions may limit this simple application of SvO2 in assessing circulatory sufficiency and cardiac output (Table 32-1). If VO2 were to increase (as occurs with exercise), hemoglobin-carrying capacity to decrease (as occurs with anemia, hemoglobinopathies, and severe hemorrhage), or SpO2 to decrease (as occurs with hypoxic respiratory failure), then for the same cardiac output, SvO
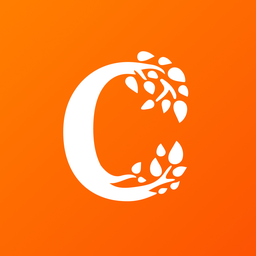
Full access? Get Clinical Tree
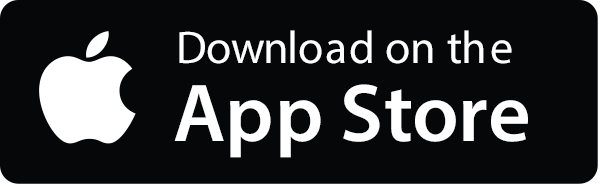
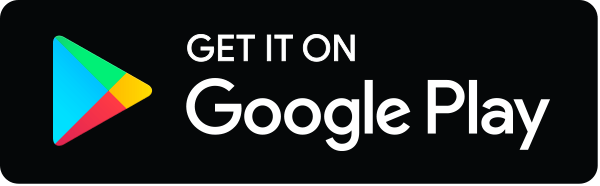
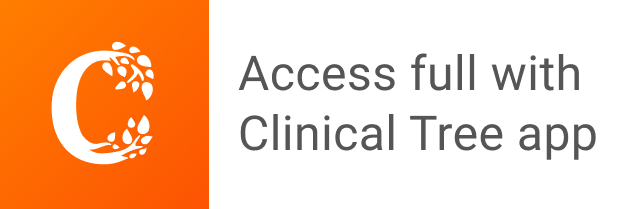