Fig. 12.1
Variation in resting metabolic rate in different types of critical patient. The graph was taken from [9]
∎
Glucose transporters (GLUT) are a group of membrane proteins that facilitate the transfer of glucose across the cell membrane, from interstitial space into cytoplasm. These carriers are usually sequestered in cytoplasmic vesicles. In humans, seven types of glucose transporters have been identified, and each one of them has a different distribution depending on the tissue. In insulin-dependent tissues and in those who directly regulate glycaemia according to variations in the level of glucose, there are three types of glucose transporters:
GLUT-4 (insulin-dependent glucose transporter)
GLUT-2 (acts as an insulin-independent glucose sensor)
GLUT-1 (responsible for the low level of basal glucose required by all cells)
In insulin-dependent tissues such as striated muscle (skeletal and cardiac muscle) and adipose tissue, the main glucose transporter is GLUT-4, which is strictly regulated by insulin concentration. The release of insulin increases both the synthesis of GLUT-4 and its translocation from the intracellular vesicles to the plasma membrane: the vesicles fuse with the membrane, and GLUT-4 transporters are inserted and become available for their function, augmenting glucose uptake from interstitial space into cytoplasm. Once glycaemia is reduced to basal concentration and insulin is cleared from the bloodstream, endocytosis sequesters GLUT-4 in vesicles. GLUT-4 concentration can also be influenced by other factors: hypercortisolaemia reduces it, while stress, physical exercise and any mechanism causing a decrease in the ATP/ADP ratio or an increase in levels of calcium ion (Ca2+), augmented blood flow and reduced levels of glycogen determine raising in GLUT-4 activity.
In skeletal muscle, GLUT-4 uptake of glucose is more intense in type I fibres (also called red fibres). Striated muscles usually take 60% of the glucose available in the bloodstream, while other tissues take the rest, and only a very small fraction goes to the adipose tissue. It has been shown that at least 80% of glucose consumption, induced by insulin, takes place in the skeletal muscle, while adipose tissue only uses 5–10% of it.
Glycogenolysis
Every day, the human organism needs to regulate a lot of different reactions and functions to preserve its balance. One of the parameters that needs to be controlled is glycaemia. When hypoglycaemia occurs, glucagon is released in the bloodstream, causing a raise in plasmatic glucose levels. Glucagon acts as a signal to the liver, leading to an increased production and release of glucose into plasma. Adrenaline induces an augmentation in glycaemia too, but, unlike glucagon, it influences mostly the muscle tissue. When these hormones bind to their receptors (both in the liver and muscle tissue), protein kinase A is activated: this enzyme starts up phosphorylase kinase. This kinase converts glycogen phosphorylase b in its activated form (glycogen phosphorylase a) by phosphorylation (while glycogen phosphorylase can be inactivated by a protein phosphatase). Glycogen phosphorylase is the first enzyme in glycogenolysis. It removes terminal glucose residue from a glycogen branch, producing glucose-1-phosphate. The latter is converted by phosphoglucomutase into glucose-6-phosphate. This can either be used for glycolysis, in muscle tissue, or be released in the bloodstream, in the liver (in hepatocytes there is a particular enzyme, glucose-6-phosphatase, which can remove the phosphate group from glucose 6-phosphate).
12.3 Starvation vs Stress [17, 20]
Unlike stress-induced metabolic alterations, the metabolic response to fasting consists in a reduction of energy expenditure (metabolic rate is reduced to 20–25 kcal/kg/day). During the first hours, the glucose stored in the liver as glycogen is depleted; after that, 90% of energy is produced by lipolysis, while proteins are used as a substrate for gluconeogenesis to synthetize glucose. This would result in a decreased protein biosynthesis, if it were not for the protein–sparing response which minimises the loss of muscle mass. Starvation induces adaptive responses that, in time, can result in malnutrition.
12.4 Hypermetabolism and Hyperdynamic Circulation
Biochemical and metabolic alterations are not the only changes caused by hypermetabolism. Also haemodynamics is altered. The augmented oxygen consumption in peripheral tissues leads to an increased cardiac output (CO) and a reduction in systemic vascular resistance (SVR). Typical clinical conditions presenting with hyperdynamic circulation are sepsis (when vasoplegia causes hypotension or dysoxic septic shock), intense adrenergic stimulation (e.g. due to postoperative pain), hyperthyroidism and hyperthermia. In a patient with hyperdynamic circulation, we should always consider underlying hypermetabolism.
In hyperdynamic circulation, the most common changes in haemodynamic parameters are increase of cardiac index and systolic volume index, possibility of hypertension or hypotension (depending on the level of vasoplegia) and reduction of systemic vascular resistance, central venous pressure and wedge pressure (changes in haemodynamic parameters in different types of shock are shown in Fig. 12.2).
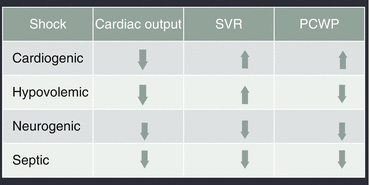
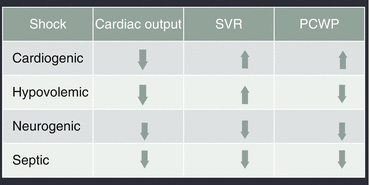
Fig. 12.2
Haemodynamic changes associated with different types of shock. Systemic vascular resistance (SVR). Pulmonary capillary wedge pressure (PCWP)
12.5 Hypermetabolism and Sepsis [5, 11, 18, 23]
Stress response is particularly emphasised in patients with sepsis: it can be induced by SIRS (systemic inflammatory response syndrome), hypermetabolism (with an increase in resting metabolic rate), hypercatabolism (protein breakdown takes place especially in muscle tissue), shock and multiple organ failure (MOF). In this case the inflammatory response can be triggered by components of the bacterial cell wall (endotoxins or exotoxins) which induce a massive release of inflammatory mediators, increasing the stress action. Augmented energy expenditure can be also explained by fever. It induces a 10–15% increase in energy expenditure for each degree Celsius elevation of temperature, even though the relation between the two parameters in critically ill patients is complicated.
We cannot address hypermetabolism and sepsis without mentioning lactate metabolism. Hyperlactatemia, due to hypoxia and hypoperfusion, is present in every low cardiac output condition, such as shock. Understanding lactatemia levels in septic shock has been shown to be a much more complex task though. In the early phase of septic shock, the clinical presentation is similar to that of cardiogenic and haemorrhagic shock. The administration of sympathomimetic drugs (adrenaline, noradrenaline, dopamine and dobutamine) causes an increase in lactatemia per se. A peculiar metabolic condition called “accelerated aerobic glycolysis” occurs in septic patients, and its main features are increased synthesis of glucose transporters across the cell membrane, augmented glycolysis (induced by endogenous and exogenous catecholamines) and inflammation. The rate of glycolysis exceeds the oxidative capacity of mitochondria: this imbalance, together with protein hypercatabolism, causes an increase in pyruvate concentration, especially in muscle cells. Pyruvate is then converted to lactate by fermentation. The increased lactate production acts as an adaptive mechanism in order to keep the carbohydrate metabolism from stopping when oxygen delivery is reduced. Lactate can be used both as an alternative fuel to glucose and a source of glucose itself. Cells keep it for producing energy and this protects from acidosis. Lactate is transported out of the cell by diffusional cotransport with protons (H+ ions), thus regulating intracellular pH. In septic shock, lactate produced in myocytes is used as a substrate in the Cori cycle (illustrated in Fig. 12.3), an effective but highly energy-consuming pathway. Lactate is transported to the liver, where it is used for gluconeogenesis: it is first converted to pyruvate and then to glucose. In this way, lactate serves to deliver additional glucose that can be used by the organism. It is also important to point out that, when the patient undergoes stress, the heart and the brain are able to use lactate as main fuel: these organs can oxidise lactate instead of glucose during anaerobic conditions, leaving glucose for other cells.
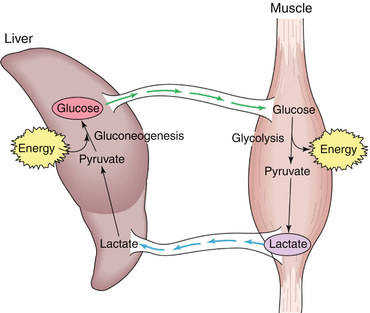
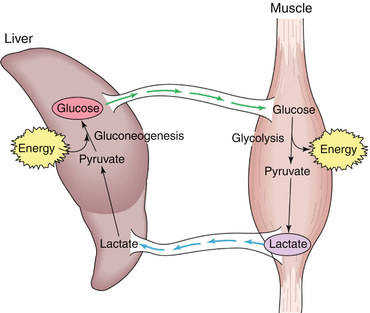
Fig. 12.3
The Cori cycle
Septic patients are extremely frail, both during the early and the late phase of illness. SIRS is a pro-inflammatory syndrome: its aim is to remove the organism responsible for the infection by increasing the activity of the immune system. When this response goes out of control or is overly prolonged, it damages the patient. Studies on septic patients have shown the existence of an anti-inflammatory response, usually occurring after SIRS, called CARS (compensatory anti-inflammatory response syndrome). CARS consists in a suppression of the immune system in order to restore homoeostasis after a severe inflammatory state. SIRS and CARS are the two sides of the same coin: each one is characterised by a particular set of cytokines, and, although they are separate responses, most of the times one follows the other (even though often they take place simultaneously with different relevance). Like SIRS, CARS can be dangerous too if it is not regulated or it fails to restore homoeostasis, making the patient vulnerable to infections. Some of the clinical features of CARS are cutaneous anergy, leucopenia, hypothermia, susceptibility to infections and failure to clear infections.
12.6 Hypermetabolism in Cancer Patients [21]
In neoplastic patients, especially at advanced stages, cachexia is frequently associated with an increased resting energy expenditure (REE). The condition is even more complicated due to the presence of symptoms such as anorexia, pain and depression, which can often cause malnutrition, both in quantitative and qualitative terms. Malnutrition affects the metabolic state of the patient, which is already impaired.
12.7 Hypermetabolism in Postoperative Patients [7, 13]
Patients undergoing surgery are an ideal model for the study of hypermetabolism caused by stress response. Surgery is in fact a clear example of a stressful event: even though anaesthesia reduces afferent neural transmission to pain centres, the organism interprets surgery as a threat. The activation of different hormonal axis causes the release of stress hormones such as cortisol, catecholamines, glucagon and growth hormone. These hormones are responsible for the condition of hypermetabolism, whose biochemical aspects have been previously discussed. This mechanism takes place in elective operations, but even more in emergency surgery, major surgery and when postoperative complications occur. The stress response purpose is the survival of the critically ill patient, by using the energy reserves and retaining water. The response becomes a harmful condition when prolonged in time. Regional and local anaesthesia has been shown to be an important factor in both reducing the stress response to surgery and influencing the postoperative outcome by bringing beneficial effects on organ function.
12.8 Hypermetabolism in Trauma Patients [4, 6, 7]
In trauma patients, the systemic inflammatory reaction is similar to the one previously described. The splanchnic region appears to be the main source of hypermetabolic response. The increased energy expenditure is not adequately matched by an implementation in blood flow, especially right after the trauma, when the patient is often hypovolaemic due to loss of blood. Hypovolaemia causes splanchnic vasoconstriction which is an important risk factor for inadequate perfusion of splanchnic region.
12.9 How Can Energy Expenditure Be Accurately Determined [1, 2, 24, 25, 26]?
Once the complex condition of the critical patient in hypermetabolic state has been analysed and understood, it is essential to think about the correct way to manage the administration of nutrients. That is important to satisfy needs and prevent negative consequences of catabolism and malnutrition. First of all, it is crucial to accurately determine energy expenditure (EE).
Historically we know two different ways to solve this problem: the use of predictive equations and the calorimetry procedure (whether direct or indirect).
Predictive equations are really manageable since no measuring instrument is required and the results can be comfortably calculated at a desk; the required data are easily available and at no great expense, either! It should not, however, be forgotten that they represent only a mere approximation of the energetic consumption of the individual being examined. Even in the analysis of healthy subjects, it is well known that predictive equations are not particularly accurate. Each of the available formulas was derived from a cohort of individuals who, potentially, could have had extremely different features from the patient that we want to examine. Let us consider, for example, the Harris-Benedict (which, when the correction factor 1.2 is applied, is the least discordant among the calorimetric measures for the male sex). This formula was worked out, in 1919, from the study of a specific population of 239 healthy adults. It is evident that the physical features of the subjects examined were dramatically different from those of people living nowadays. Changes in lifestyle, working conditions, services and facilities available, alimentary regimen and disposition to physical exercise are only some of the differences we can find between these two different groups.
Secondly, even when resorting to these formulas, we have to choose which one, from the large number available, is the most suitable for our patient. There are indeed differences that may reach 20–30% of the estimated value when choosing one rather than another (Table 12.1). It has been proven that the use of these equations can frequently lead to underfeeding (in formulas that underestimate the EE) or to overfeeding (in equations that overestimate the EE) the patient concerned. This can, obviously, compromise the patient’s prognosis. Considering the extreme variations of energy expenditure in the different conditions of stress that can occur in a critical patient, it is obvious how only the correct measurement of how much energy (EE) the patient actually consumes, and not the estimate of how much the patient should theoretically consume, can represent the baseline for the institution of a correct caloric supply.
Table 12.1
Comparison of REE values obtained by indirect calorimetry and with the different predictive equations in 49 elderly male subjects who were being mechanically ventilated
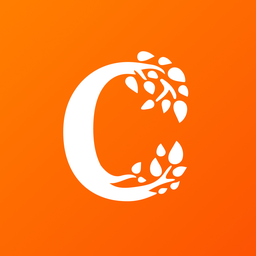
Full access? Get Clinical Tree
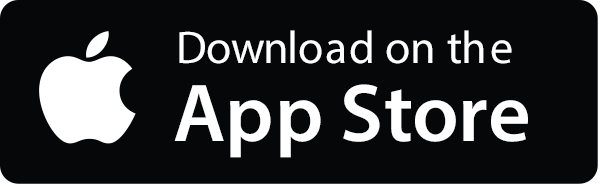
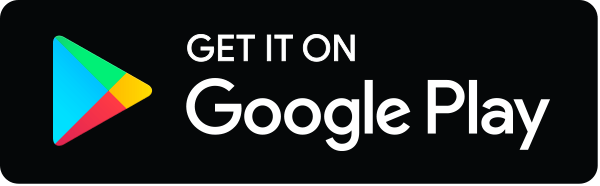
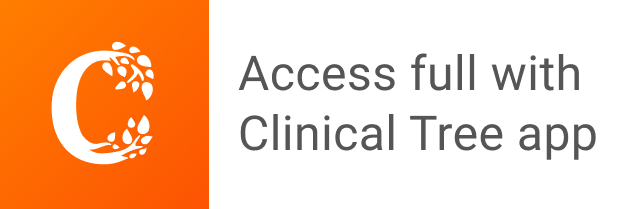