Abstract
This chapter reviews the pharmacology of vasopressors and inotropes used commonly in acute care settings. It focuses on the pharmacodynamic properties of the drugs to a greater degree than their pharmacokinetic properties as most have short half-lives, are administered by continuous infusion, and are titrated to clinical effect. Clinicians know that when treating patients experiencing severe hypotension or cardiac failure, the effects of vasopressors and cardiotonic drugs depend on many associated factors, including acid-base status, temperature, blood volume, and concomitant drug administration. These factors are reviewed and recommendations are made for the rational selection and application of relevant vasopressors and inotropes.
Keywords
vasopressors, inotropes, vasoactive drugs, pharmacology
This chapter reviews the pharmacology of vasopressors and inotropes used commonly in acute care settings and comparable new drugs with promising clinical potential. It focuses on the pharmacodynamic properties of the drugs to a greater degree than their pharmacokinetic properties because most of these drugs have short half-lives, are administered by continuous infusion, and are titrated to clinical effect.
Relying on landmark studies from the past as well as recent findings, this chapter seeks to build the scientific foundation on which the clinical use of these agents is based. Because their application to human pharmacology is unreliable, data derived exclusively from animal studies are not considered. Even when only human data are considered, the effects of vasopressors and inotropes vary substantially because of patient factors. Clinicians know that when treating patients experiencing severe hypotension or cardiac failure, the effects of vasopressors and cardiotonic drugs depend on many associated factors, including acid-base status, temperature, blood volume, and concomitant drug administration.
Historical Perspective
Vasoactive drugs have an extensive history and have been in clinical use for millennia. The early identification and isolation of vasoactive substances was based on extraction from plants and endocrine glands. For instance, ephedrine has been in clinical use as a diaphoretic and circulatory stimulant for more than 5000 years as the active component of the Chinese drug ma huang. Until the drug was finally isolated in 1887, it was extracted from the plant Ephedra sinica .
Similarly, foxglove had been in use for hundreds of years; William Withering published his historic book An Account of the Foxglove, and Some of Its Medical Uses in 1785. This text detailed Withering’s work with extracts of the plant Digitalis purpurea and described effects and side effects of the drugs now known as digoxin and digitoxin .
In the late 17th century, it was recognized that “an extract of the suprarenal glands caused contraction of the arteries and led to an increase in the beat of the auricles and ventricles,” and that an extract of the pituitary gland possessed vasopressor activity. These substances would eventually be named epinephrine and vasopressin .
While early medicinal chemistry work focused on developing progressively purer isolates of the active substances from natural sources, it eventually shifted to synthesizing drugs chemically. Dopamine was first synthesized in 1910 by Barger and Ewins, who immediately recognized its potency as a vasopressor. Vasopressin was the first polypeptide hormone successfully synthesized, for which du Vigneaud won the Nobel Prize in Chemistry in 1955. Work by von Euler confirmed that norepinephrine helped mediate the activity of the sympathetic nervous system and contributed to his 1970 Nobel Prize.
In the modern era, attempts were made to develop drugs with specific characteristics. Dobutamine was synthesized in the early 1970s for the specific purpose of providing a high level of inotropy without the vasodilatory limitations of isoproterenol. Similarly, milrinone was developed in the early 1980s as an alternative to amrinone but without the high incidence of fever and thrombocytopenia that limited the use of amrinone.
The development of novel vasopressors and inotropes continues to this day. Levosimendan, for instance, entered clinical use in Europe as recently as 2000 and numerous drugs are currently under investigation around the world.
Structure-Activity Relationships
Many of the drugs in this chapter share structural similarities that affect their pharmacologic actions, although a few are chemically unrelated ( Fig. 25.1 ). Many sympathomimetics are derived from the parent compound β-phenylethylamine. Many of these drugs are also referred to as catecholamines due to the presence of hydroxyl substitutions on carbons 3 and 4 of the benzene ring of β-phenylethylamine. The most basic example of a catecholamine is dopamine, which is the 3,4-hydroxyl substituted form of βphenylethylamine. It is the metabolic precursor to both norepinephrine and epinephrine as the substrate for dopamine β-hydroxylase. The addition of an N-substitution increases the activity at β-adrenergic receptors. Norepinephrine, like epinephrine, is derived from β-phenylethylamine, but the lack of N-substitution decreases its activity at the β receptors. The impact of the degree of amino substitution on β-receptor activity is further reflected in the structures of isoproterenol and dobutamine. Both of these drugs have bulky side chains and as such have a high degree of β specificity.

Phenylephrine and ephedrine are not considered catecholamines, in that they are not hydroxylated on both the 3 and 4 carbons of their benzene ring (phenylephrine has a single substitution and ephedrine has none). This lack of hydroxylation prevents phenylephrine from effectively binding the β receptor despite the N-methyl substitution. Ephedrine’s lack of hydroxylation substantially decreases its ability to stimulate directly adrenergic receptors. The presence of a methyl group on the α-carbon of ephedrine blocks oxidation by monoamine oxidase and prolongs its action.
Milrinone, vasopressin, and levosimendan neither share structural similarities with the sympathomimetic drugs discussed nor with one another. Milrinone is a bipyridine methyl carbononitrile derivative of amrinone. Vasopressin, as a nonapeptide hormone, consists of a sequence of nine amino acids (Cys-Tyr-Phe-Gln-Asn-Cys-Pro-Arg-Gly), whereas levosimendan is a pyridazone-dinitrile derivative.
Mechanisms
Although the drugs discussed in this chapter are applied in similar clinical settings, they do not all share a pharmacologic class or mechanism. They are perhaps best classified and considered based on their mechanism for increasing inotropy and vasoconstriction (see Chapter 23 ). Although the drugs that increase inotropy do so by different mechanisms, the common endpoint is positively influencing the interaction of the calcium ion (Ca 2+ ) with actin and myosin in the cardiac myocyte ( Fig. 25.2 ). Each of the β agonists, phosphodiesterase inhibitors, cardiac glycosides, and calcium sensitizers accomplishes this in a different way.

Drugs that act on the β 1 receptor (such as epinephrine, dobutamine, dopamine, isoproterenol, and, to a lesser extent, ephedrine and norepinephrine) begin by stimulating the receptor on the cardiac myocyte sarcolemma with subsequent activation of the G s protein. This protein activates adenylyl cyclase and enhances the formation of cyclic adenosine monophosphate (cAMP), which activates protein kinase A, thereby phosphorylating and increasing the open probability of voltage-gated Ca 2+ channels. These channels allow Ca 2+ influx to increase cytosolic Ca 2+ concentration, which activates the coupling of actin and myosin in the myocyte. Protein kinase A also activates a Ca 2+ -adenosine triphosphatase (ATPase) on the sarcoplasmic reticulum, leading to increased Ca 2+ uptake in diastole and improved lusitropic function.
The inotropic effects of phosphodiesterase inhibitors (e.g., milrinone), like those of the adrenergic agonists, are mediated by cAMP. Unlike adrenergic agonists that increase cAMP by stimulating adenylyl cyclase, milrinone inhibits the breakdown of cAMP by phosphodiesterase type III (PDE3). Increased cAMP enhances Ca 2+ release from the sarcoplasmic reticulum and increases the force generated by actin-myosin. The vasodilatory action of milrinone is also cAMP mediated. In vascular smooth muscle, cAMP inhibits myosin light chain kinase, the enzyme responsible for phosphorylating myosin light chains and causing smooth muscle contraction. Inhibition of PDE3 increases cAMP, thereby promoting vascular smooth muscle relaxation.
Digoxin increases cytosolic Ca 2+ by inhibiting the action of a sodium-potassium adenosine triphosphatase (Na + ,K + -ATPase) on the cell membrane of cardiac myocytes. This leads to an increase in cytosolic sodium ion (Na + ), thereby decreasing the activity of Na + -Ca 2+ exchange and indirectly resulting in an increase in intracellular Ca 2+ available to interact with actin and myosin.
Levosimendan, referred to as a calcium sensitizer , has a mechanism that is fundamentally different from the other inotropes discussed herein. Rather than increasing the content of intracellular Ca 2+ , it acts to modulate the interaction of Ca 2+ . It first binds the N-terminal lobe of cardiac troponin C (TnC), thereby stabilizing the Ca 2+ -bound form of the protein. This prolongs the systolic interaction between actin and myosin and increases the force of contraction. Because binding of levosimendan to TnC is dependent on the cytosolic Ca 2+ concentration, it occurs almost exclusively during systole, leaving diastolic function relatively unaffected. Importantly, unlike other drugs discussed in this chapter, the increased inotropy is achieved without an increase in myocardial oxygen demand.
The majority of drugs discussed in this chapter exert their vasoconstrictive actions via α 1 receptors in the vasculature; the exception is vasopressin that acts on the V1 receptor. Stimulation of α 1 or V1 receptors on vascular smooth muscle act (via separate G proteins) to stimulate phospholipase C (PLC), which hydrolyzes phosphatidylinositol bisphosphate (PIP 2 ) to generate inositol trisphosphate (IP 3 ) and diacylglycerol (DAG). IP 3 increases Ca 2+ release from the sarcoplasmic reticulum, while DAG activates protein kinase C to increase Ca 2+ influx via voltage-gated Ca 2+ channels. This increase in cytosolic Ca 2+ increases vascular smooth muscle tone.
Metabolism/Pharmacokinetics
Vasopressors and inotropes generally have short half-lives and are rapidly metabolized, are administered by continuous infusion, and are titrated to clinical effect. This means that for practical purposes these drugs are pharmacokinetic equals; thus pharmacokinetic factors do not typically play an important role in rational drug selection of a specific inotrope or vasopressor. In general, these drugs exert their effects with an ongoing infusion; the effects rapidly decrease once the infusion is terminated. Levosimendan is a notable exception to this general rule.
The catecholamine class of drugs, which includes epinephrine, norepinephrine, dopamine, dobutamine, and isoproterenol, are all rapidly inactivated by methylation of a hydroxyl group of the catechol structure by catechol- O -methyltransferase (COMT). In addition, monoamine oxidase (MAO) catalyzes oxidative deamination of this group of compounds (with the exception of dobutamine). Approximately 25% of dopamine is converted to norepinephrine in adrenergic nerve terminals; these nerve terminals also take up norepinephrine. Even though phenylephrine is not a catecholamine, it is nonetheless metabolized by MAO. Ephedrine and milrinone largely resist metabolism and are excreted in the urine, whereas vasopressin is metabolized by specific vasopressinases in the liver and kidney.
Levosimendan is unique in this group of drugs, in that it is metabolized to active compounds that are eliminated slowly. This results in clinical effects for up to a week after discontinuation of an infusion.
Pharmacodynamics and Drug Interactions
The pharmacodynamic profile of specific inotropes and vasopressors is a function of their relative receptor activities and mechanisms; an overview of receptor activities and physiologic effects is presented in Table 25.1 . Adrenergic receptors have traditionally been divided into α and β, and have been subdivided into α 1 , α 2 , β 1 , β 2 , and β 3 . Further subtyping has been performed, and several genetic variations have been described (see “Pharmacogenetics”).
Drug | α Receptor | β 1 Receptor | β 2 Receptor | Cardiac Output | Heart Rate | SVR | MAP | PVR |
---|---|---|---|---|---|---|---|---|
Epinephrine | ++ | ++ | ++ | ↑ | ↑ | ↑ | ↑ | 0 |
Isoproterenol | 0 | +++ | +++ | ↑ | ↑ | ↓ | ↓ | 0 |
Norepinephrine | +++ | ++ | 0 | 0 | 0 | ↑ | ↑ | ↑ |
Dopamine | ++ | ++ | 0 | ↑ | ↑ | ↑ | ↑ | 0 |
Dobutamine | 0 | +++ | + | ↑ | ↑ | ↓ | ↓ | ↓ |
Milrinone | 0 | 0 | 0 | ↑ | 0 | ↓ | ↓ | ↓ |
Phenylephrine | +++ | 0 | 0 | 0 | ↓ | ↑ | ↑ | ↑ |
Vasopressin | 0 | 0 | 0 | 0 | 0 | ↑ | ↑ | 0 |
Ephedrine | + | + | + | ↑ | ↑ | ↑ | ↑ | 0 |
Levosimendan | 0 | 0 | 0 | ↑ | 0 | ↓ | ↓ | ↓ |
The predominant location of α 1 receptors is on peripheral vasculature; stimulation results in vasoconstriction of the skin, muscles, and renal and mesenteric vasculature. There is some contribution of peripheral α 2 receptors to vasoconstriction, but agonism of α 2 receptors is not a major characteristic of drugs discussed here (for pharmacology of α 2 agonists, see Chapter 10 ).
β 1 Receptors are primarily located in the heart, where their stimulation results in increased inotropy, chronotropy, and lusitropy. β 2 Receptors are widely distributed through the vasculature. Stimulation in peripheral vasculature results in dilation of muscular, splanchnic, and renal vessels. Bronchial smooth muscle has a high concentration of β 2 receptors, the activation of which causes bronchodilation. Additional effects include stimulation of glycogenolysis in the liver and a slowing of peristalsis. The β 3 receptor has been known for years to exist in adipose tissue, where its stimulation results in lipolysis. Its existence in the heart has been more recently recognized, and its role in normal physiology and disease, as well as the pharmacologic implications, is still being investigated. Current thinking suggests that β 3 -receptor agonism in the heart causes a decrease in inotropy.
There is a potential interaction between monoamine oxidase inhibitors (MAOIs) or tricyclic antidepressants (TCAs) and several inotropes and vasopressors ( Table 25.2 ). Because MAO contributes to the metabolism of norepinephrine and TCAs inhibit its reuptake, patients taking either type of drug can have an exaggerated hypertensive response to norepinephrine, drugs that enhance norepinephrine release (ephedrine and dopamine), and drugs that are metabolized by MAO. This adverse pharmacokinetic drug interaction can have important implications in the perioperative and intensive care settings.
Drug | Drug of Choice | Bolus Dose | Infusion Dose | Relevant Drug Interactions |
---|---|---|---|---|
Epinephrine | Anaphylaxis; cardiac arrest | 5–10 µg, up to 1 mg for cardiac arrest | 0.02–0.3 µg/kg per minute | β-blockers, MAOIs, proarrhythmic medications |
Isoproterenol | Refractory bradycardia | No bolus dosing | 0.01–0.2 µg/kg/min | No co-infusion with alkaline medications |
Norepinephrine | Septic shock | No bolus dosing | 0.05–0.5 µ/kg per minute | MAOIs, TCAs |
Dopamine | Septic shock with systolic dysfunction | No bolus dosing | 1–20 µg/kg per minute | MAOIs, TCAs, butyrophenones, phenothiazines, phenytoin |
Dobutamine | Stress echocardiography | No bolus dosing | 2–20 µg/kg per minute | Co-administration with alkaline solutions can decrease activity |
Milrinone | Weaning from cardiopulmonary bypass | Loading dose: 20–50 µg/kg over 10 min | 0.2–0.75 µg/kg per minute | Can precipitate with furosemide |
Phenylephrine | Mild hypotension from general or regional anesthesia | 50–200 µg | 20–200 µg/min | MAOIs, TCAs |
Vasopressin | Post–cardiopulmonary bypass vasoplegia | 0.5–2 units for mild hypotension, 20 units | 0.01–0.04 µ/min | Carbamazepine, TCAs, norepinephrine, lithium, heparin |
Ephedrine | Mild hypotension from general or regional anesthesia | 5–10 mg | No infusion dosing | MAOIs, TCAs |
Levosimendan | Unclear at this time | Loading dose: 12 µg/kg over 10 min | 0.05–0.2 µg/kg per minute | None yet identified |
Pharmacogenetics
Although basic knowledge of the α- and β-adrenergic receptors forms the foundation for understanding the pharmacology of inotropes and vasopressors, recent research has unveiled considerable genetic complexity in the receptors. There are at least nine distinct receptor subtypes (three subtypes of each α 1 , α 2 , and β) that are expressed in a variety of tissues. Many of these receptor subtypes also have well-described genetic variants. For example, 12 single-nucleotide polymorphisms (SNPs) have been identified in the β 1 receptor and 19 in the β 2 receptor. These are simple variations in the genetic code, but it is believed that they translate into clinically significant phenotypes. Polymorphisms have also been identified in the α 1 and α 2 receptors. There appears to be an association between some of these genotypes and the development of hypertension and heart failure.
The majority of research on the impact of adrenergic receptor genetic variation has focused on its implications on the development and treatment of cardiovascular disease, as well as on the clinical outcomes after certain cardiac diagnoses (e.g., myocardial infarction). Little work has focused on the effects of vasopressors and inotropes in these different genotypes. It is reasonable to expect, however, that clinically significant differences seen in the response to receptor antagonists (e.g., β blockers) might also be observed for receptor agonists. Indeed, a polymorphism in the β 1 receptor affects the response to dobutamine, with a significantly greater heart rate and inotropic response. Even though much work remains to be done in this area, it is likely that at least some of the large interindividual variability seen in the response to these drugs is a function of genetic variation.
Individual Drugs
Epinephrine
Epinephrine is a naturally occurring sympathomimetic with nonselective adrenergic agonist activity. It is synthesized, stored, and released by the chromaffin cells of the adrenal medulla in response to physiologic stress. It binds to α, β 1 (the predominant β receptor in the heart), and β 2 (the predominant ββ receptor in the lungs and vasculature) receptors. Action at the β 3 receptor is not currently a target of clinical application of epinephrine.
Epinephrine is the drug of choice in two extreme clinical conditions: anaphylactic shock and cardiac arrest ( Fig. 25.3 ). In anaphylaxis, α-receptor–mediated vasoconstriction of small arterioles and precapillary sphincters increases mean arterial pressure (MAP) and decreases mucosal edema. Its β-receptor–mediated effects cause bronchodilation and stabilization of mast cells. The latter decreases the release of histamine, tryptase, and other inflammatory mediators that perpetuate the pathophysiology of anaphylaxis. In cardiac arrest, epinephrine is given in large doses (1 mg every 3–5 minutes) to increase MAP, thereby increasing cerebral perfusion pressure during chest compressions. The value and safety of its β-receptor–mediated effects during cardiac arrest are controversial because they increase myocardial oxygen consumption. However, studies demonstrate better survival with epinephrine than without it.

Other indications for epinephrine take advantage of specific subsets of its nonselective adrenergic agonism profile. Epinephrine is used to treat asthma (β 2 -mediated bronchodilation), severe hypotension associated with bradycardia (β 1 -mediated chronotropy) and/or low cardiac output (β 1 -mediated inotropy), and to prolong the effects of local anesthetics (α-mediated vasoconstriction). Low doses of epinephrine (0.02–0.05 µg/kg per minute) are used to increase depressed cardiac output after cardiopulmonary bypass; other catecholamines and inotropes have similar effects, but none has proven superior to epinephrine in terms of patient outcome. Epinephrine has also been studied as an alternative to other vasopressors in the treatment of vasodilatory shock from sepsis, even though the data do not yet support its use as a first-line therapy.
Epinephrine’s effects are route, time, and dose dependent. At low doses (0.01–0.05 µg/kg per minute), the β-receptor effects of epinephrine predominate, while at higher doses, α effects predominate (see Table 25.1 ). An intravenous bolus of epinephrine (5–15 µg) causes an initial increase in heart rate, systolic blood pressure, and systemic vascular resistance (SVR, from stimulation of α and β receptors), and a subsequent decrease in systolic and diastolic blood pressure and vascular resistance (from continued stimulation of β receptors with peripheral vasodilation.)
In healthy subjects, increasing rates of epinephrine infusion (0.01–0.2 µg/kg per minute) progressively increase heart rate and systemic blood pressure. In general, at progressively higher continuous infusion rates, heart rate, blood pressure, SVR, and cardiac output increase while pulmonary artery pressure, central venous pressure, and pulmonary artery occlusion pressure remain unchanged.
Mast cell stabilization and bronchodilation via stimulation of β 2 receptors are the two most important nonhemodynamic, therapeutic effects of epinephrine. Epinephrine has numerous other nonhemodynamic effects that are potentially adverse. At doses typically administered for vasopressor and/or inotropic effects, these potentially adverse effects include the following:
- •
Hyperglycemia—due to increased liver glycogenolysis, reduced tissue uptake of glucose, and inhibition of pancreatic secretion of insulin
- •
Hypokalemia—due to increased uptake of K+ in skeletal muscle secondary to stimulation of β 2 receptors. Infusion of epinephrine at a rate of 0.1 µg/kg per minute reduces plasma K + concentration by about 0.8 mEq/L.
- •
Lactic acidosis—in theory due to inhibition of pyruvate dehydrogenase, causing pyruvate to be shunted to lactate. Although the mechanism is not fully elucidated, epinephrine infusion results in lactic acidosis even in the absence of tissue hypoxia and may not necessarily signify a poor prognosis.
- •
Myocardial ischemia—due to hypertension, tachycardia, and increased inotropy that increase myocardial oxygen demand.
Epinephrine is administered by continuous infusion, bolus, infiltration, or inhalation. Usual intravenous infusion doses are 0.02 to 0.3 µg/kg per minutes. Intravenous bolus doses range from 5 to 10 µg for moderate hypotension (MAP 40–60 mm Hg) unresponsive to other vasopressors up to 1 mg as recommended by the American Heart Association guidelines for cardiac arrest. The usual intramuscular dose is 0.3 mg administered into the lateral thigh (vastus lateralis), which produces significantly higher plasma concentrations than administration into the deltoid or subcutaneously. Subcutaneous administration results in delayed absorption and lower peak plasma concentrations than other routes. This is generally reserved for treatment of severe asthma in doses of 0.3 to 0.5 mg for adults or 0.01 mg/kg for children, when inhaled selective β 2 agonists cannot be administered. In addition, epinephrine can be administered via an endotracheal tube during cardiac arrest if other routes are not available; the recommended dose is double the intravenous dose diluted with 10 mL of normal saline solution. Epinephrine is not effective orally owing to rapid metabolism and does not cross the blood-brain barrier in sufficient amounts to directly affect the central nervous system.
Epinephrine should not be used in patients with acute cocaine intoxication because of the potential for exacerbation of myocardial ischemia and stroke. In patients with dynamic obstructions to ventricular outflow (e.g., tetralogy of Fallot and hypertrophic obstructive cardiomyopathy), epinephrine can worsen outflow obstruction and lower cardiac output. Administration of epinephrine with a β blocker can lead to significant α-receptor stimulation without opposing β-receptor–mediated vasodilation, which can result in severe vasoconstriction, hypertension, and heart failure. Care should also be taken when administering epinephrine with medications that predispose the heart to arrhythmia, particularly digitalis and halothane.
Isoproterenol
Isoproterenol was approved by the U.S. Food and Drug Administration (FDA) in 1947 and was used initially to treat asthma. Interestingly, it was the first drug for which the FDA required a package insert beginning in 1968. As the isopropyl derivative of norepinephrine, isoproterenol is a synthetic sympathomimetic with nonselective β-adrenergic activity.
Stimulation of cardiac β 1 receptors by isoproterenol increases heart rate, inotropy, and lusitropy, resulting in an increase in cardiac output and systolic blood pressure. Stimulation of β 2 receptors results in vasodilation of the muscle, kidney, skin, and splanchnic circulations, thereby decreasing total peripheral vascular resistance and mean and diastolic blood pressure. The decrease in systemic blood pressure combined with increases in myocardial contractility and heart rate can precipitate myocardial ischemia in patients with significant coronary artery disease. At higher doses, palpitations, headache, and flushing can occur.
Isoproterenol was used initially via inhaler to treat asthma and bronchospasm but has been replaced by β 2 -selective bronchodilators. Currently isoproterenol is indicated in hemodynamically significant bradycardia until cardiac pacing can be established. In prior years, it was used immediately following cardiac transplantation to enhance inotropy and chronotropy without concomitantly increasing systematic vascular resistance. Currently other drugs are used in this setting more commonly (e.g., epinephrine, milrinone, and vasopressin with cardiac pacing as necessary). Isoproterenol is also being used during electrophysiology procedures to stimulate the underlying arrhythmia for better mapping and increases the likelihood of a successful ablation.
Norepinephrine
Norepinephrine is a naturally occurring sympathomimetic with both α- and β 1 -receptor affinity. Its effects are comparable to epinephrine on β 1 receptors, but because of its greater affinity for α receptors and its near-total inactivity at β 2 receptors, it is an intense vasoconstrictor. As the primary neurotransmitter of the sympathetic nervous system, it is released from postganglionic sympathetic nerve endings and represents 10% to 20% of the catecholamine content of the adrenal medulla.
Norepinephrine causes an increase in systolic and diastolic blood pressures owing primarily to an increase in SVR (see Table 25.1 ). Cardiac output does not increase and can decrease owing to increased resistance to ventricular ejection. Heart rate remains unchanged or decreases from compensatory baroreceptor-mediated vagal activity. Blood flow decreases in renal, mesenteric, splanchnic, and hepatic beds. Norepinephrine increases pulmonary vascular resistance (PVR), probably by α 1 -mediated vasoconstriction.
Norepinephrine is the drug of choice for septic shock when MAP is less than 65 mm Hg despite adequate volume resuscitation. Compared with dopamine in sepsis, norepinephrine is more likely to improve hypotension with fewer arrhythmias and tachycardia. Norepinephrine is also used to treat hypotension following cardiopulmonary bypass. However, when used to treat hypotension associated with milrinone administration, norepinephrine is less effective than vasopressin in preserving a beneficial ratio of systemic and PVR. Although norepinephrine increases PVR, its ability to substantially increase right ventricular perfusion pressure can make it a useful vasopressor in right heart failure.
With regard to adverse effects, norepinephrine can cause severe hypertension with increased myocardial workload and cardiac ischemia. Systemic vasoconstriction can impair perfusion of the gut and other organs, resulting in organ dysfunction and metabolic acidosis. Clinical studies, however, have not consistently shown a decrease in splanchnic perfusion or worsening organ function when patients with sepsis are treated with norepinephrine. In some cases a decrease in splanchnic perfusion is associated with improved gastrointestinal perfusion, suggesting a redistribution of blood flow in the gut.
Dopamine
Dopamine is a naturally occurring catecholamine that stimulates β 1 -and α 1 -adrenergic receptors, as well as vascular D 1 -dopamine receptors (primarily in mesenteric and renal vasculature). It is synthesized in the kidney and has both diuretic and natriuretic effects. In addition to its peripheral actions, it is an important neurotransmitter in the central nervous system (see Chapters 8 and 12 ).
At low plasma concentrations, dopamine acts primarily on the D 1 receptor in renal, mesenteric, and coronary vasculature (see Table 25.1 ) to produce vasodilation of these beds with a resultant increase in glomerular filtration rate, renal blood flow, Na + excretion, and urine output. Low doses can also decrease SVR. Higher doses directly stimulate β 1 receptors and enhance release of norepinephrine from sympathetic nerve terminals to increase myocardial contractility, heart rate, systolic blood pressure, and pulse pressure. Diastolic blood pressure is minimally affected but PVR can increase. At high doses, stimulation of α 1 receptors predominates, resulting in generalized peripheral vasoconstriction.
It is commonly stated that doses of 0.5 to 3 µg/kg per minute stimulate primarily DA1 receptors, 3 to 10 µg/kg per minute stimulate primarily β 1 receptors, and greater than 10 µg/kg per minute primarily stimulate α receptors, but clinically the hemodynamic effects of dopamine are difficult to predict based on these empirical dosing guidelines. In healthy male volunteers, weight-based dopamine administration resulted in up to 75-fold intersubject variability in plasma concentrations. However, no study has yet compared plasma concentrations of dopamine with its effects. Therefore dosing should be titrated to physiologic effect, rather than being based on rigid concepts of relative receptor activity for a given dosage.
Dopamine had previously been recommended as a first-line treatment for septic shock with systolic dysfunction. Recent studies showing worse outcomes with dopamine have resulted in a change in recommendations and it is now suggested only in patients at low risk for arrhythmia or those with bradycardia. Comparison of dopamine with norepinephrine for treatment of shock showed a higher incidence of arrhythmias in all patients and higher mortality in patients with cardiogenic shock treated with dopamine. Compared with dobutamine after cardiac surgery and in patients with chronic heart failure, dopamine resulted in less hemodynamic improvement. When compared with dopexamine, it resulted in significantly more adverse cardiac events.
Renal dose dopamine refers to an infusion of dopamine in low doses (usually 1–3 µg/kg per minute) for treatment or prevention of acute renal failure with a goal of selective stimulation of D 1 receptors. It is a misleading phrase and outdated concept, as the effects of dopamine even in low doses are not exclusively limited to the kidneys. Even though low doses of dopamine increase renal blood flow, glomerular filtration, and urine output, numerous studies have failed to show a decreased incidence of renal failure with its use.
Dopamine can cause tachycardia, tachyarrhythmias, and myocardial ischemia, and at high doses causes decreased splanchnic perfusion and gut ischemia. In addition to its hemodynamic effects, dopamine reduces the ventilatory response to hypoxemia, consistent with its role of as a neurotransmitter in the carotid bodies. Dopamine infusions alter endocrine and immune function, including decreased secretion of growth hormone, prolactin, and thyroid stimulating hormone. Like other vasoconstrictors, dopamine can cause skin necrosis and sloughing if extravasation occurs.
The renal and mesenteric vasodilating properties of low-dose dopamine are suppressed by dopamine receptor antagonists such as butyrophenones and phenothiazines. There are reports of dopamine causing hypotension and bradycardia in patients taking phenytoin.
Dobutamine
Dobutamine is a direct-acting synthetic catecholamine and is the drug of choice for the noninvasive assessment of coronary disease (dobutamine stress echocardiography). Dobutamine is also used for short-term treatment of congestive heart failure and for low cardiac output after cardiopulmonary bypass. In patients with chronic low output cardiac failure, dobutamine was superior to dopamine in its ability to increase cardiac output without untoward side effects. Similarly, it is superior to dopamine in managing hemodynamically unstable patients after cardiac surgery, reducing cardiac filling pressures and PVR with less trachycardia. Compared with milrinone after cardiac surgery, dobutamine was “comparable,” producing a greater increase in cardiac output, blood pressure, and heart rate but with a higher incidence of arrhythmias.
In patients with congestive heart failure, the principal effect of dobutamine is an increase in myocardial contractility and ventricular ejection mediated by its β 1 effects. In contrast to epinephrine or dopamine, dobutamine generally reduces SVR by a combination of direct vasodilation and a reflex decrease in sympathetic vascular tone. This might be offset by the increase in cardiac output, leading to no change or a decrease in MAP. Dobutamine generally decreases cardiac filling pressures and PVR. Dobutamine has a variable effect on heart rate, but it can significantly increase heart rate (particularly at the higher concentrations used in stress echocardiography). After cardiopulmonary bypass the primary mechanism of increased cardiac output by dobutamine is an increase in heart rate (approximately 1.4 beats/min per microgram per kilogram per minute) with an increase in SVR. The contrasting results of these studies reflect dobutamine’s complex mechanisms of action, particularly with regard to the balance of α 1 stimulation and inhibition by its isomers, as well as patient factors.
Dobutamine can produce tachycardia, arrhythmias, and hypertension. Dobutamine can exacerbate myocardial ischemia in susceptible patients by increases in heart rate and contractility.
Milrinone
Milrinone is a phosphodiesterase type III inhibitor, and as such is a synthetic noncatecholamine inodilator. Milrinone increases cardiac index with reductions in arterial pressure, left ventricular end-diastolic pressure, and PVR. Heart rate can increase, although this is not a consistent response and bradycardia can also occur. Compared with dobutamine, milrinone produces less tachycardia with more pulmonary and systemic vasodilation.
Milrinone significantly increases success in the first attempt at weaning from cardiopulmonary bypass with less need for catecholamine support but with a greater requirement for additional vasoconstrictors. Milrinone might be preferable to adrenergic agonists in patients with chronic heart failure undergoing cardiopulmonary bypass as downregulation of adrenergic receptors in this population can lead to decreased responsiveness to catecholamines. In addition, milrinone improves flow in grafted internal mammary artery and saphenous vein grafts. Intravenous milrinone has also been used to reverse cerebral vasospasm after subarachnoid hemorrhage, while inhaled milrinone has been used to treat severe pulmonary hypertension and acute lung injury.
Milrinone is attractive for use in right heart failure by increasing ventricular contractility and decreasing PVR. However, milrinone-induced decreases in SVR and arterial blood pressure might offset these benefits and worsen supply-demand balance in the failing right heart. For this reason, milrinone is often combined with norepinephrine or vasopressin in an attempt to offset peripheral vasodilation. Comparing these two combinations, adding low-dose vasopressin to milrinone might be superior to adding norepinephrine in improving the ratio of systemic to PVRs.
Inhaled milrinone offers the potential advantage of decreased PVR and increased ventricular contractility while maintaining SVR and MAP. Its use has been shown to improve the ratio of arterial oxygen tension to fraction of inspired oxygen (Pa o 2 /F io 2 ) as well as decrease the intrapulmonary shunt fraction (Qs/Qt). When administered during cardiopulmonary bypass, inhaled milrinone may be superior to intravenous administration in reducing pulmonary reperfusion syndrome by preventing endothelial dysfunction in the pulmonary arterial system.
The most common adverse effect of milrinone is arterial hypotension, though this is often a desired effect. Milrinone use is an independent risk factor for the development of atrial fibrillation after cardiac surgery, but the incidence is less than with dobutamine. About 12% of patients given milrinone in phase II and III trials developed ventricular arrhythmias (primarily premature ventricular contractions).
Milrinone is typically given intravenously, but it can also be nebulized. Intravenous dosing of milrinone is initiated with a loading dose of 20 to 50 µg/kg over 10 minutes, followed by an infusion of 0.2 to 0.75 µg/kg per minute. Owing to the high degree of renal clearance, the dose should be reduced in patients with reduced creatinine clearance. Inhaled milrinone is nebulized in a concentration of 1 mg/mL. The most common administration regimen has been a single dose of 5 mg, though longer infusions have been studied as well.
Phenylephrine
Phenylephrine is a synthetic noncatecholamine α 1 agonist and produces dose-dependent vasoconstriction of cutaneous, muscular, mesenteric, splanchnic, and renal vasculature (see Table 25.1 ). Systemic arterial vasoconstriction increases systolic, diastolic, and MAPs, with reflex bradycardia. Phenylephrine can also cause pulmonary vasoconstriction and pulmonary hypertension.
Phenylephrine is the drug of choice for initial treatment of mild hypotension with normal or increased heart rate in the setting of general or regional anesthesia. The use of phenylephrine to support blood pressure during spinal anesthesia for cesarean section has long been discouraged because of concerns that vasoconstriction could have a deleterious effect on placental blood flow. Several studies have strongly contradicted this traditional teaching by documenting that phenylephrine does not worsen fetal outcome and might in fact be superior to ephedrine. The use of phenylephrine in the management of septic shock is generally not recommended. Exceptions to this include cases in which norepinephrine causes significant arrhythmia or when vasodilation is refractory to other thrapy. Phenylephrine has been used to increase right ventricular perfusion in pulmonary hypertension and right heart failure, though it can worsen right ventricular function and raise pulmonary artery diastolic pressures; norepinephrine appears to be more effective in this setting. Phenylephrine is used topically as a nasal decongestant; as a mydriatic; and in ear, nose, and throat surgeries to constrict mucosa or control bleeding.
Severe bradycardia or even brief asystole can occur with higher doses of phenylephrine. With left ventricular dysfunction, the combination of bradycardia and increased afterload can significantly reduce cardiac output. Case reports document the risk of pulmonary edema, arrhythmias, cardiac arrest, and death when phenylephrine is used topically in excessive doses during head and neck surgery to control bleeding. Topical doses should be limited to no more than 0.5 mg in adults, and blood pressure and heart rate should be monitored. Severe hypertension can require treatment with an α 1 antagonist such as phentolamine or with a direct vasodilator such as hydralazine. β Blockers or calcium channel blockers should not be administered in this setting because their cardiac depressant effects can result in acute heart failure.
Vasopressin
Arginine vasopressin (AVP, vasopressin, also known as antidiuretic hormone) is a nonapeptide hormone synthesized in the magnocellular neurons of the paraventricular and supraoptic nuclei of the hypothalamus (see Chapter 36 ). It is stored and released from neurosecretory vesicles in the posterior pituitary gland (neurohypophysis).
Vasopressin is given typically by continuous intravenous infusion. Previously recommended infusion rates for hypotension were from 0.01 to 0.04 units/min based on a study that suggested increased cardiac complications with doses above 0.04 units/min. While some evidence suggests that a dose of 0.067 units/min results in better cardiovascular function with no increase in side effects, the surviving sepsis campaign recommends a maximal dose of 0.03 units/min in patients with sepsis and suggests that any further increase should be done only as salvage therapy. Further study is required to identify the optimal doses for different clinical settings. Vasopressin can be given as a bolus of 1 to 2 units to treat intraoperative hypotension, though its effects are short-lived.
The hemodynamic effects of vasopressin are complex and vary depending on the presence or absence of intact sympathetic and renin-angiotensin systems. Interestingly, the effect of vasopressin infusions in healthy volunteers appears to be minimal even at high plasma concentrations. This paradoxical finding can be explained by the action of vasopressin on the area postrema of the central nervous system. The expected vasoconstrictive effect is effectively counterbalanced by an augmented baroreflex inhibition of efferent sympathetic activity. In patients with septic shock, low-dose vasopressin increases systemic arterial blood pressure and vascular resistance, but it does not alter pulmonary vascular resistance and pressures, cardiac filling pressures, or cardiac index. Heart rate can decrease, though this finding is not consistent.
Even though it is not considered a first-line therapy, vasopressin is used as an adjunct to catecholamines in the treatment of septic shock. Patients with septic shock have much lower plasma vasopressin concentrations than those with cardiogenic shock. This has been interpreted as a relative vasopressin deficiency caused by early depletion of hypothalamic stores or inhibition of vasopressin release. The Vasopressin and Septic Shock Trial (VASST) compared norepinephrine with low-dose vasopressin to norepinephrine alone. There was no difference in overall mortality or adverse events, but mortality was reduced in patients with less severe sepsis who were given vasopressin.
Guidelines on the use of vasopressin in cardiopulmonary resuscitation are evolving. Endogenous vasopressin levels are higher in patients who are successfully resuscitated. Studies have compared the use of vasopressin and epinephrine in cardiac arrest with variable outcomes. It does not appear that vasopressin confers a significant benefit compared with epinephrine, and the most recent American Heart Association guidelines have removed vasopressin as a single agent to replace a dose of epinephrine.
Cardiopulmonary bypass is normally associated with a substantial increase in circulating vasopressin. In some cases of postbypass hypotension, plasma vasopressin concentrations are inappropriately low. These patients frequently respond to low doses of vasopressin, as do some patients with vasodilatory shock after cardiac transplantation or left ventricular assist device placement.
Although not supported by specific studies, vasopressin is also used to treat intraoperative hypotension during general or epidural anesthesia. Clinical experience suggests that it might be useful in treating hypotension refractory to catecholamines in patients receiving long-term treatment with drugs that inhibit the renin-angiotensin system (angiotensin-converting enzyme inhibitors and angiotensin receptor blockers).
In patients with septic shock, vasopressin reduces gastrointestinal mucosal perfusion and increases liver enzymes and total bilirubin concentrations. In addition, it decreases platelet count (likely due to increased platelet aggregation) but does not significantly alter coagulation.
Numerous drugs interact with vasopressin. Potentiation of its antidiuretic effect can be seen with carbamazepine, chlorpropamide, clofibrate, fludrocortisone, and TCAs. Inhibition of the antidiuretic effect can be seen with demeclocycline, norepinephrine, lithium, heparin, and alcohol.
Ephedrine
Ephedrine is a synthetic noncatecholamine agonist at α, β 1 , and β 2 receptors with both direct and indirect actions. Ephedrine is given as an intravenous bolus of 5 to 10 mg. It is effective in the same dose range when administered intramuscularly, albeit with slower onset and longer duration. When given in repeated doses, tachyphylaxis occurs, probably because of depleted norepinephrine stores. Ephedrine causes an increase in systolic, diastolic, and MAPs. It increases myocardial contractility, heart rate, and cardiac output (see Table 25.1 ).
In the acute care setting, ephedrine is used primarily to treat mild hypotension and bradycardia associated with general or regional anesthesia. Previously, ephedrine was the first-line therapy for parturients with hypotension secondary to spinal or epidural anesthesia based on studies in pregnant ewes suggesting that ephedrine preserved uterine blood flow compared with other vasopressors. These data have been challenged recently; phenylephrine appears to be as good or better in preserving uterine blood flow and does not cause or worsen maternal tachycardia.
At higher doses, ephedrine causes hypertension and tachycardia. Because it crosses the blood-brain barrier, ephedrine can cause agitation and insomnia. In patients with prostatic hypertrophy, ephedrine can produce urinary retention. Because ephedrine causes release of norepinephrine, patients taking MAOIs can have an exaggerated hypertensive effect.
Digoxin
Digoxin, a cardiac glycoside, exerts its positive inotropic effects by inhibiting the plasma membrane Na + ,K + -ATPase of cardiac myocytes. This leads to an increase in available Ca 2+ as described earlier. Although digoxin has been in clinical use for hundreds of years as an inotrope and to control heart rate in atrial fibrillation, it has largely been replaced by more effective medications with fewer side effects.
Digoxin is currently indicated (as a second- or third-line therapy) for ventricular rate control in atrial fibrillation and in the treatment of systolic heart failure. Although it is effective in providing symptomatic relief for heart failure, it does so with a significant increase in mortality and its use should essentially be considered palliative. It is likewise associated with increased mortality in patients with atrial fibrillation, and although it is effective in decreasing ventricular rate at rest, it does not prevent exercise induced tachycardia, does not aid in conversion to sinus rhythm, and may be associated with conversion from sinus rhythm back to atrial fibrillation.
In addition to concerns about increased mortality with the use of digoxin, its use is significantly limited by the high incidence of side effects. The therapeutic index of digoxin is very small and requires plasma concentration monitoring, and its use is frequently associated with a wide variety of cardiac arrhythmias, including sinus bradycardia, sinus arrest, atrioventricular conduction delays, second- or third-degree heart block, and malignant ventricular arrhythmias. Digitalis toxicity is generally treated with digitalis binding antibody, as well as lidocaine, magnesium, phenytoin, and correction of hypokalemia.
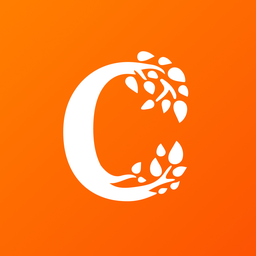
Full access? Get Clinical Tree
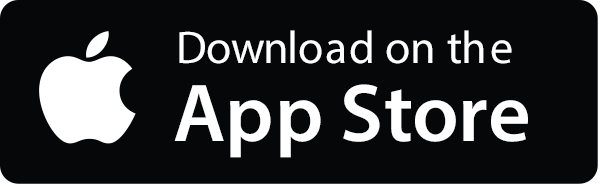
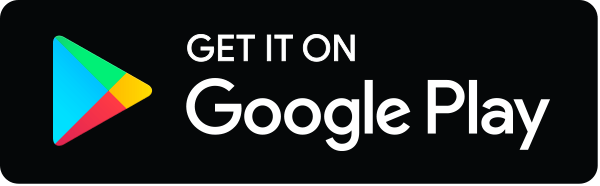