Chapter 92 Ultrasound and Telemedicine in the Wilderness
For online-only figures, please go to www.expertconsult.com
It is beyond the scope of this chapter to provide detailed descriptions of all ultrasound techniques or of the huge range of telemedicine devices. Excellent texts are dedicated purely to telemedicine or to clinical ultrasound.59,63,90 Our goal is to provide sufficient detail so that a provider, even with little or no formal training in ultrasound, can acquire and begin to apply potentially lifesaving techniques while in the field. Experimental studies and routine clinical practice have established that these skills can rapidly, safely, and effectively be learned for use in austere environments, both by medical experts and nonphysicians. A growing body of evidence reveals that ultrasound images of diagnostic quality can be acquired by untrained nonphysicians under the real-time guidance of ultrasound experts thousands of miles away.65 With these examples in mind, we provide simple instructions and representative images for various clinical ultrasound techniques that are likely to prove critical in austere environments. We also discuss multiple field-based research techniques for which ultrasonographic imaging is especially well suited and for which there is great promise.
Ultrasound
Ultrasonographic imaging devices promise to revolutionize wilderness medical care. Wilderness travelers now have access to machines that are lightweight, compact, and sufficiently robust to be carried in a daypack, and outputs that can be interpreted in the field (Figure 92-1, online). Commercially available ultrasound machines readily provide diagnostic imaging of organs under austere conditions.

FIGURE 92-1 The SonoSite 180 is a simple, portable ultrasound device well suited to austere environments.
(Copyright N. Stuart Harris.)
Sound frequencies employed for ultrasound imaging are well in excess of those perceived by humans (20-20,000 Hz). Frequencies of 2 to 20 megahertz (MHz) may be used. The technology underlying ultrasound imaging predates Wilhelm Konrad Röntgen discovery of x-rays (1895) by more than a decade.17,75 In 1942, Dussik21 described the utility of ultrasound for human medical imaging. Early machines were massive, cumbersome, and produced images limited in detail when compared with the crisp images of early radiographs. During the 1960s and early 1970s, ultrasound technology evolved to the point that it began being used for clinical care. Obstetrics and cardiology were early adopters. Clinical use of ultrasound by European emergency care providers grew rapidly throughout the late 1970s, and by their American counterparts in the late 1980s. Education in ultrasound imaging is now a mandated skill for many nonradiology physician-training programs. A component of sonographic imaging, Focused Assessment With Sonography for Trauma (FAST examination), is now recommended for early use by the Advanced Trauma Life Support (ATLS) guidelines developed by the American College of Surgeons.
Sonographic imaging is based on the principle of the piezoelectric effect, a property defined by Pierre and Jacques Curie in 1880. They described the piezoelectric effect as a property of quartz crystals that creates an electric potential when stimulated by a mechanical force. In corollary, these same crystals produce a mechanical potential when an electric force is applied.17 Using these properties, a modern ultrasound machine functions by applying an electric charge to a piezoelectric crystal (the transducer or probe) that then converts the electric signal to a sound wave. Structures within the body reflect these sound waves back toward the piezoelectric crystal of the probe. The crystal turns this mechanical force into an electric current, which is quantified and interpreted to produce an image.
Ultrasound for Wilderness Clinical Care And Research
Advantages
Portability
Extraordinarily powerful ultrasound equipment can be readily carried by hand. As compared with other diagnostic imaging devices that might be considered for wilderness use (such as conventional radiography, computed tomography [CT] or magnetic resonance imaging [MRI]), ultrasound machines are much more compact, lighter, and more portable (Table 92-1). On rare occasions, when a full-sized ultrasound machine may be required, it can be transported to a road-accessible field site or hypobaric research chamber, which is not the case with CT or MRI. The revolution in digital and ultrasound technology and manufacturing has allowed small laptop-sized machines to overtake the capabilities of older, larger machines for virtually every application. Because portable machines have become small (often weighing less than 8 to 12 lb), their utility has increased for wilderness settings. In austere locations with no power source (e.g., remote alpine environment or postdisaster area), ultrasound devices can be powered using batteries, including portable photovoltaic arrays. A SonoSite MicroMaxx ultrasound machine used successfully on the 2007 Caudwell Xtreme Everest expedition at Camp 4 on the South Col (8016 m [26,299 feet]) of Mt Everest was powered by standard MicroMaxx batteries charged at base camp and Camp 2 using a combination of generators or solar power.8 We have conducted research using a SonoSite 180 machine carried to the summit of Mt Kilimanjaro (5895 m [19,341 feet]) powered only by hand-carried solar arrays. A portable ultrasound setup with batteries, power source, laptop computer for image storage and backup, and enough ultrasound gel for clinical use or research can reasonably be expected to weigh approximately 13.6 kg (30 lb) and be carried to remote locations by a single physician or investigator. Some machines (Terason Ultrasound) are essentially modular attachments to laptop computers, further reducing the need for separate pieces of equipment. Prototypes exist that can fit in the palm of the hand; it is inevitable that miniaturization and power system improvements will continue and become increasingly affordable.
TABLE 92-1 Advantages and Limitations of Ultrasound in the Wilderness
Advantages of Ultrasound | Limitations of Ultrasound |
---|---|
Portable, lightweight, field-ready | Electronic equipment—sensitive to cold, dust, trauma |
Safe, nonionizing (allows multiple assays) Relatively inexpensive Provides immediate data | Power system (or numerous batteries) required for extended backcountry use |
Requires operator to learn techniques | |
Easy to learn techniques, or to be guided from a distance using telemedicine | |
Allows multiple organ systems to be investigated | |
Excellent flexibility for both research/clinical care | |
Allows novel research investigations |
Safety/Noninvasiveness
Diagnostic ultrasound has few known risks.31 To highlight this fact, ultrasound is the clinical imaging modality of choice for many delicate high-risk patient populations, including pregnant women and their fetuses. Ultrasound is routinely used at the bedside for rapid assessment of critically ill trauma and medical patients. A physician with a bedside ultrasound can quickly and in real time assay for a range of common, acute life threats, including intraperitoneal free fluid or pericardial effusion, obviating the risks associated with delays due to patient transport or image processing. No other clinical imaging system allows this combination of speed, safety, portability, and immediacy of result.
In many research applications, such as replacement of invasive pulmonary arterial catheterization by transthoracic echocardiography, ultrasound provides an essentially risk-free, noninvasive option to take the place of a potentially risky, invasive technique.3 The safe, noninvasive, and painless nature of ultrasound compared with other diagnostic and research techniques encourages patient compliance and aids recruitment for research protocols by making participation more attractive to potential subjects. Importantly, for research purposes, the U.S. Department of Health and Human Services’ Office for Human Research Protections specifically identifies ultrasound, Doppler measurements, and echocardiography as research methodologies eligible for expedited investigational review board review, significantly easing administrative burdens and potentially shortening the time between conception and execution of studies. For research purposes, because ultrasound itself does not significantly impact subjects or other experimental manipulations, sonographic monitoring can easily be added to other experimental protocols for purposes of collecting additional relevant data (such as monitoring additional parameters of potential interest during a drug trial) or conducting a separate parallel study. For example, we joined research conducted by U.S. Army Research Institute of Environmental Medicine collaborators and were able to assay sonographically measurable parameters (e.g., optic nerve sheath diameter [ONSD] and pulmonary arterial pressure) during a study originally designed to examine the effects of moderate altitude prepositioning on combat readiness of military recruits during exposure to high altitude. Ultrasound does not employ ionizing radiation, so there is no known additive risk of repeated ultrasound exposures.
Limitations
There are some general drawbacks of ultrasound for wilderness clinical and high-altitude research use.92 Although portable and relatively durable for in-hospital clinical use, ultrasound machines can be fragile and require careful packing and handling during transport and field use. Wilderness use can place strains on ultrasound machines far in excess of what the machines ordinarily encounter in routine clinical practice. Mechanical failure has jeopardized or terminated wilderness clinical/research expeditions.9,28,40,89
To enhance effectiveness and protect the ultrasound device, it may be necessary to sleep with batteries next to the body, prepare warm water in which to soak a probe to achieve reliable functioning in the cold, or to operate the machine within a plastic bag to protect against dust. The risk for theft (particularly in chaotic, urban, or postdisaster settings) is real. Traditional (spinning) hard drives have been implicated in machine failure at high altitude, probably due to cold and the influence of marked decreased barometric pressures on internal air-filled cushioning components.13 Solid-state memory devices (i.e., flash cards) without moving parts are readily available for primary data storage on laptop computers and USB drives. These solid-state units should prove to be significantly more reliable than their mechanical counterparts. A plan for rapid servicing or exchange of machines in case of malfunction should be made in advance. Bringing a backup machine is an effective hedge against malfunction. Thorough testing before field use, careful machine handling, device security, and thoughtful attention to potential site-specific problems enable a clinician or researcher to increase the likelihood of maintaining the ultrasound unit during a wilderness experience.
Before departure, sufficient ultrasound training must be completed to ensure that diagnostic-quality images and accurate measurements can be reliably obtained. Although some imaging techniques, such as echocardiography, require months of intensive training, other applications, such as thoracic, long-bone, and abdominal ultrasound imaging, are straightforward and have been taught successfully in brief sessions, or even through remote expert guidance at the time of image acquisition. A growing body of evidence, both on Earth and in space, confirms that these techniques can be effectively taught to nonphysicians with minimal prior training when guided by audiovisual linkage to expert ultrasonographers.65
Introduction to Clinical Imaging
Several excellent resources discuss the physics and theoretic underpinning of ultrasound.58,59,63 We now address the practical topics of “gain” and “depth” controls (Figure 92-2, online). Clinicians may think of gain as analogous to volume on a radio. By turning up gain, the “brightness” of signals displayed on the screen is increased. This can be useful to adequately view an image on a screen under sunny conditions. Different machines have different methods for adjusting depth. Depth controls the distance under the probe that is displayed on the screen. Depth adjustments allow the user to maximize the size of the anatomic structure of interest within the viewing area. For example, to locate a subcutaneous foreign body, depth typically should be very limited (2 to 3 cm [0.8 to 1.2 inches]) so that fine detail may be appreciated in this superficial structure. In the case of deeper tissue imaging (e.g., a subxiphoid view of the heart or examination of the aorta in an obese adult), depth might need to be markedly increased (16 to 20 cm [6.3 to 7.8 inches]). B-mode stands for brightness and is the typical display mode with which clinicians and patients are familiar. B-mode produces a two-dimensional image. M-mode stands for motion and displays a representation of motion within a single anatomic plane over a linear axis of time. It can be effectively used to highlight and quantify the movement of structures (e.g., physiologic lung sliding or fetal heart rate). Probes (transducers) can vary in two critical manners: frequency (high or low) and shape (linear or curved). The probe qualities preferred for each of the techniques we describe are shown in Table 92-2.
TABLE 92-2 Types and Ideal Uses of Different Ultrasound Probes
Probe Type | Ideal Uses |
---|---|
Low-frequency (2-5 MHz) curved array | FAST examination Thoracic evaluation (for pulmonary edema) |
(Excellent depth of imaging) | |
Cardiac views | |
High-frequency (8-10 Mhz) linear array | Foreign body locationThoracic assessment (for pneumothorax) |
(Excellent resolution, limited depth) | |
Eye evaluation, optic nerve sheath assays | |
Fractures |
FAST, Focused Assessment With Sonography for Trauma.
Common Clinical Imaging Techniques
Focused Assessment with Sonography for Trauma (fast)
The FAST examination consists of four standard views:
To obtain this view, place the probe in the midline just superior to the symphysis pubis (Figure 92-9). Angle the probe toward the rectum. Then with probe held in place, slide and rotate up the spine until the pouch of Douglas or rectovesical space is visualized. A negative image shows each gender-specific space without evidence of hypoechoic free fluid (Figure 92-10). A positive image reveals perivesicular free fluid (Figure 92-11).
Thoracic Ultrasound for Pneumothorax and Pulmonary Edema
An additional technique to screen for the presence of pneumothorax can be accomplished using M-mode scanning. Two characteristic findings may help determine presence or absence of a pneumothorax. To visualize these findings, the ultrasound machine must have an M-mode setting (this typically is available on even rudimentary devices). M-mode imaging produces a linear representation of movement in a single plane over a period of time. By aligning that plane through the structure of interest, changes within that plane (e.g., lung sliding or fetal heart rate) can be readily appreciated. To obtain thoracic images, the linear, high-frequency probe is placed on the anterior chest, in the 2nd to 3rd intercostal space, starting at the anterior axillary line and moving toward the costal margin. Using M-mode imaging, presence or absence of lung sliding results in characteristic images. Normal sliding movement between parietal and visceral pleura will produce the “waves on the beach” sign (Figure 92-18). Normal skin and intercostal muscles have minimal movement during breathing and appear as flat, stacked lines using M-mode. Below the bright white line of the pleural surface, normal lung tissue moves, which results in this structure having a granular appearance. This image has been described as reminiscent of waves (the superficial/superior, stacked, linear portion of the image) against a beach (the deeper/lower granular portion of the image). In patients with a pneumothorax, the lung tissue appears stationary and so appears in M-mode as a series of stacked lines, just like the superficial muscle above it. This results in the “bar code” sign (Figure 92-19).
Ultrasound may also be used to assay for pulmonary edema, using the comet-tail technique. (Figure 92-20). Monitoring of pulmonary edema is of interest both for typical congestive heart failure with related pulmonary edema and for high-altitude pulmonary edema (HAPE). Thoracic ultrasound assays allow visualization of severity of edema in overt HAPE and allow monitoring for subclinical forms that may affect oxygenation at high altitude. Conventional radiography has proven insensitive to subclinical pulmonary edema and early HAPE and does not allow easy tracking of accumulation or resolution of pulmonary edema.37,88 Techniques that overcome these shortcomings, such as CT or MRI, are not available in the field. A series of papers describes use of ultrasound for identification and monitoring of pulmonary edema in hospitalized patients.1,46 We described its use for diagnosis and monitoring of HAPE.23–26 Use of this technique for high-altitude clinical use and research, particularly for the monitoring of subclinical edema, requires further study; ease of performance and early results are encouraging. This technique requires a low-frequency (2.5 to 5 MHz), curved “cardiac” probe. The chest is scanned in the 2nd to 5th intercostal spaces on the right and the 2nd to 4th intercostal spaces on the left in the midaxillary, anterior axillary, midclavicular, and parasternal lines (28 total fields) (Figure 92-21). The total number of comet tail artifacts—defined as echogenic, coherent, wedge-shaped signals with a narrow origin in the near field of the image, arising from the pleural line and extending to the edge of the screen (Figure 92-22)—are tallied to yield a total comet-tail score that correlates with the degree of pulmonary edema. These findings can also be accurately referred to as B-lines. This examination can be reliably completed in less than 5 minutes.46
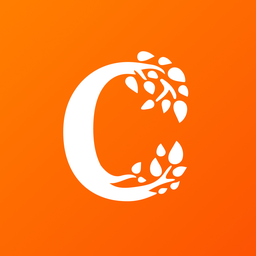
Full access? Get Clinical Tree
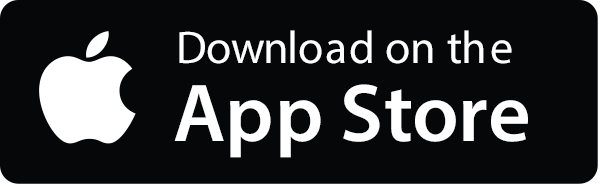
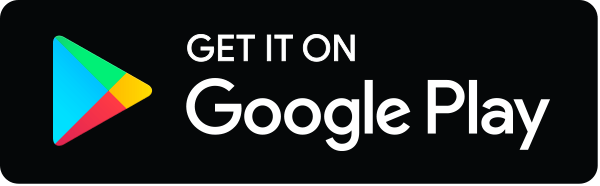