The rib cage is formed by twelve pairs of ribs which are posterior connected to the thoracic vertebrae of the vertebral column. At the anterior side of the thorax the sternum fixes the upper seven ribs. The lower ribs are either connected indirectly to the sternum or are attached to muscles and the abdominal wall (so-called floating ribs). The ribs are interconnected with each other by the internal and external intercostal muscles. As the connections of the ribs to the vertebrae, the intercostal muscles, and the sternum are flexible, the rib cage represents a quite stiff though deformable cover of the internal organs and facilitates respiration.
While the rib cage is very flexible in a newborn, its stiffness increases with growth but still retains a certain flexibility. In the elderly the joints between the ribs and the sternum and the vertebrae, respectively, become stiffer. In addition, the ribs become more brittle due to changes of the bone properties. This increases the likeliness of rib fractures and reduces the protective potential of the rib cage.
The interior volume covered by the rib cage can be divided into three areas. The right and left outer regions contain the lungs. The centre section, called mediastinum, hosts among others the heart, the trachea and large vessels.
The left lung consists of two lobes, while the right lung consists of three lobes. Two layers of membranes surround the lung: the visceral pleura, which encloses the lung tissue, and the parietal pleura, which covers the entire inside of the rib cage (including the cranial side of the diaphragm and the vertebral bodies). The visceral and the parietal pleura are not connected to each other, but form a small cavity. This pleural cavity is an enclosed space. To keep the lung in their inflated state, a continuous underpressure is maintained in the pleural cavity. If this underpressure cannot be maintained (for example due to a perforation of the chest), the lungs deflate and the pleural cavity is filled with air. This phenomenon is called pneumothorax (see Sect. 5.2.2).
For respiration the diaphragm, the rib cage and the intercostal muscles function as a pump by drawing air into the lung (inspiration) and expelling air from the lung (expiration). For inspiration the thoracic volume is increased by lifting the rib cage and by lowering the diaphragm. Consequently the lung will expand and air is sucked in. To normally expel the air (expiration) the thoracic structures and the diaphragm are relaxed.
The mediastinum is located between the two lungs, the thoracic vertebrae and the sternum. Large vessels included are the aorta, vena cava, the pulmonary arteries and veins (Fig. 5.1). Due to the restricted space available in the mediastinum, a compression of the anterior rib cage may easily cause injuries to internal structures.
5.2 Injury Mechanisms
This description of thoracic injuries and the according injury mechanisms focuses on blunt impacts in traffic accidents. Hence only scenarios where a flat or blunt object strikes the chest without penetration are regarded. This type of impact is most often seen in automotive accidents with an occupant contacting, for example, the steering wheel, the dashboard or components of restraint systems. Special attention has to be given in this context to the problem of car occupants who are seated in an unusual position (“out-of-position”, e.g., front passengers keeping the feet on the dashboard) during a crash situation. Likewise, elderly passengers are of concern (Yoganandan et al. 2007).
If the thorax is suddenly decelerated due to a blunt impact, three different injury mechanisms can be distinguished: compression, viscous loading, and inertial loading of the internal organs. Furthermore, any combination of these three basic phenomena can occur.
The resulting injuries can be categorised as skeletal injury and soft tissue injury. Most often the thoracic wall and the lung are injured together with rib fractures, fractures of the sternum, and pleura ruptures. In case of fractures of the vertebral column, injuries of the spinal cord may also occur, possibly leading to transverse lesion (in case of motorcyclists this sometimes results in quadriplegia). Fortunately such injuries are less frequently recorded, as are injuries on the aorta, the heart, the oesophagus and the diaphragm. Table 5.1 provides an overview of different injuries and their according AIS (Abbreviated Injury Scale) rating.
AIS skeletal injury | AIS soft tissue injury | ||
---|---|---|---|
1 | One rib fracture | 1 | Contusion of bronchus |
2 | 2–3 rib fractures; sternum fracture | 2 | Partial thickness bronchus tear |
3 | 4 or more rib fractures on one side; 2–3 rib fractures with hemothorax or pneumothorax | 3 | Lung contusion; minor heart contusion |
4 | Flail chest; 4 or more rib fractures on each of two sides; 4 or more rib fractures with hemo- or pneumothorax | 4 | Bilateral lung laceration; minor aortic laceration; major heart contusion |
5 | Bilateral flail chest | 5 | Major aortic laceration; lung laceration with tension pneumothorax |
– | – | 6 | Aortic laceration with hemorrhage not confined to mediastinum |
To date, the mechanisms of rib fractures and some of the lung injuries are reasonably well understood whereas some mechanisms leading to other injuries of the thoracic organs still merit further research. Figure 5.2 summarises possible soft tissue thoracic injuries.
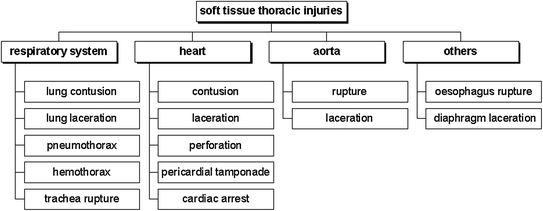
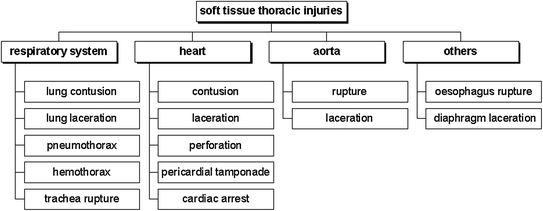
Fig. 5.2
Possible soft tissue thoracic injuries
5.2.1 Rib Fractures
According to the AIS, a single rib fracture can be graded as AIS 1. If 2-3 ribs are broken, the grade increases to AIS 2. Hence this type of injury is usually not severe and most single rib fractures are in fact self-healing. However, if multiple fractures occur, life threatening complications may arise. If the skin and the soft tissue overlaying the fracture remain intact, the fracture is called a closed fracture. If, on the other hand, sharp edges of broken ribs perforate the chest wall, the fracture is called an open fracture. Such open fractures are of particular concern because they can lead to a pneumothorax, lung collapse and infections. Broken ribs may also perforate the visceral or parietal pleura, causing respiratory problems.
Generally, sagittal loading of the thorax is more likely to cause single rib fracture, while lateral impact more often results in multi rib fracture. In principle, ribs can fracture at any point, but most likely they break at the point of maximum curvature or at the location where a force is applied. Hence, given the fact that the ribs are stronger curved laterally, together with fewer muscle tissue that covers and thus protects the ribs in that area, lateral fractures are more likely. The site of lateral rib fracture(s) depends on the shape of the impacting body (Fig. 5.3).
In case of multi rib fracture the thorax wall may lose its overall stability. This can result in a thorax motion that is contrary to normal: during inspiration the disrupted thorax wall is sucked in and thus reduces the volume of the lung. On expiration the thorax wall moves outwards making it difficult to expel the air out of the lung. The greater the area of thorax wall damaged, the lesser the amount of air which can be exchanged. This condition is called a flail chest which eventually results in hypoxemia.
According to cadaver studies (e.g. Stalnaker and Mohan 1974; Melvin et al. 1975) the number of rib fractures depends on the magnitude of rib deflection rather than on the rate of deflection. Due to the viscous nature of the thorax, the amount of force, however, depends on the rate at which the force is applied. Hence, force appears to be related to the number of rib fractures for a given loading rate. Plastic deformation properties of bone may furthermore have an important influence on peak stresses in ribs (see Sect. 2.2). Whenever at a certain location the stress reaches a plastic limit, there is no further (or only a minimal) stress increase until a final failure level is attained. If plastic deformation processes are disregarded, therefore, stresses may be overestimated.
The occurrence of rib fractures is strongly age dependent. While the rib cage can be compressed frontally in a young person until it contacts the spine without fracturing a rib (but compressing the organs in between), the rib cage of individuals of more than 50 years of age break at much lower loads, often, for example, during the cardiac rescue procedure.
5.2.2 Lung Injuries
As indicated in Fig. 5.2, injuries to the respiratory system mainly concern lung injuries. Due to thorax compression (both with and without rib fracture) a lung contusion can occur. This often happens in combination with a flail chest.
Unlike rib fractures, lung contusion is rate dependent (Fung and Yen 1984). At high velocities, a compression or pressure wave is transmitted through the thorax wall to the lung tissue, causing damage to the capillary bed of the alveoli. Sometimes also central lung contusion without damage of the surrounding tissue is observed. As a serious complication, lung contusion also increases the risk of pneumonia, i.e. an inflammation of the lung tissue.
Laceration and sometimes also perforation of the lung tissue can be observed at sites of rib fractures. This may result in a pneumothorax or a hemothorax. In the first case the pleural cavity is filled with air, in the second case with blood. A combined situation where the pleural cavity contains both blood and air is called a hemo-pneumothorax.
A pneumothorax results from a perforation of the pleura, i.e. a hole is created in the pleural sac between the lung and the rib cage, caused, for instance, by broken ribs. On inspiration the intrapleural pressure is reduced and air is sucked into the pleural cavity through the leak in the lung. During expiration the laceration in the lung tissue is compressed preventing the air in the pleural cavity to be expelled. Hence, while breathing, the amount of air inside the pleural cavity increases, eventually compressing the lung.
A hemothorax also reduces the effective lung volume, but due to blood in the pleural cavity. Hereby a laceration of blood vessels (e.g. in the lung tissue) may cause blood to accumulate in the pleural cavity.
5.2.3 Injuries to Other Thoracic Organs
From thoracic impact, the heart can be subjected to several injuries including contusion and laceration (Fig. 5.2). Contusion occurs due to compression and depends on the associated velocity, while laceration may be due to high magnitude of compression over the sternum. At high rates of loading, the heart may undergo arrhythmia, fibrillation or arrest. High speed blunt impacts (15–20 m/s) appear to interrupt the electromechanical transduction of the heart wall—a condition called commotio cordis (Maron and Estes III 2010). Figure 5.4 illustrates a thoracic impact, with the heart under compression between the sternum and the vertebral column.
Furthermore, major thoracic blood vessels like the aorta may be injured. Rupture and laceration are the most likely mechanisms resulting from blunt trauma to the thorax. Cavanaugh (2002) reports that arterial injuries account for 6–8 % of AIS > 2 only, but represent 27–30 % of the estimated harm. It is also remarkable that 80–85 % of the victims sustaining an aortic trauma in an automotive accident die at the scene of the accident (e.g. Smith and Chang 1986; Butler et al. 1996). Mechanisms of injury were found to be predominately high speed motor vehicle crashes followed by falls and pedestrians being struck (e.g. Ochsner et al. 1989).
Aortic rupture is thought to occur either from traction or shear forces generated between relatively mobile portions of the vessel and points of fixation or, secondly, due to direct compression over the vertebral column or, thirdly, caused by an excessive sudden increase of intraluminal pressure. Aortic rupture after thorax compression is shown schematically in Fig. 5.5. Additionally, Viano (1983) reported that the inertial loading of the blood-filled heart can cause the heart to displace in the thoracic cavity and thus stretch points of attachment of the aortic arch, such as the superior arteries or the ligamentum arteriosum. This may occur if the heart is displaced vertically, laterally, or obliquely. Further, Viano considered the possibility of aortic laceration in combination with hyperextension of the neck in high speed loading (Fig. 5.6). As for the site of thoracic aortic injury, it was found that the region of the aortic isthmus, just distal to the origin of the left subclavian artery, is most vulnerable. It accounts for the vast majority of such injury (e.g. Butler et al. 1996; Creasy et al. 1997).
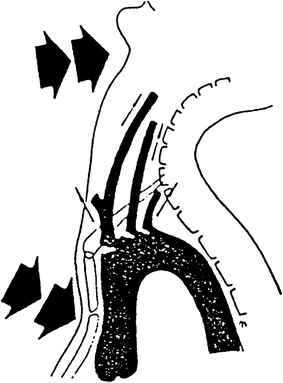
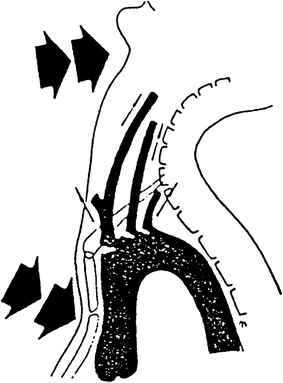
Fig. 5.6
Thorax compression in combination with hyperextension of the neck can result in laceration of the aorta (adapted from Viano 1990)
5.3 Biomechanical Response
Many biomechanical tests have been performed under controlled laboratory conditions to measure the biomechanical response of the human thorax in terms of accelerations, forces, deformations and pressures. In particular cadaver tests, extensively conducted in the 1970s, were performed to obtain details of resulting injury to the body after impact. The data was then used to develop frontal and side impact dummies as well as to develop injury criteria. Furthermore the data was used to establish and validate mathematical models of the thorax.
In terms of test conditions, mainly pendulum and sled tests were used. Additionally, quasi-static tests—some with volunteers—were performed to determine the stiffness of the thorax.
5.3.1 Frontal Loading
To investigate the biomechanical response of the thorax to frontal loading, extensive test series were performed. Human cadavers were impacted in pendulum tests using a 6-inch-diameter rigid pendulum (Fig. 5.8). Measuring the deflection of the sternum, force-deflection characteristics for the thorax were determined (e.g. Kroell et al. 1971, 1974; Stalnaker and Mohan 1974). Figure 5.9 shows a representative force-deflection curve as obtained from such experiments. The hysteresis curve can be divided into a loading and an unloading phase. The loading phase is characterised by an initial rapid rise which is mainly due to the viscous properties of the thorax, and a plateau region that is also due to a viscous response. At maximum deflection, the impactor and the test subject are moving at a common velocity. The forces measured at this point are due to inertial forces caused by whole-body acceleration, and the elastic forces due to tissue compression. The unloading phase of the curve represents the unloading of the compressed tissues and follows the elastic non-linear unloading of the thorax. Analysing the relationship between the force plateau and impactor velocity, it was found that the force plateau increases with impactor velocity except for impactors with low mass but high velocity, which do not at all exhibit a force plateau. Furthermore, it was shown that lower impactor masses resulted in lower deflections (Lobdell et al. 1973).
Based on such cadaver tests, force-deflection corridors for different combinations of impactor mass and velocity were developed which are used for performance requirements for dummies.
In addition to dynamic pendulum tests focusing on sternal impacts, quasi-static tests have been performed. Since three-point belts and airbags are frequently used today, lower rate loading has become more important in frontal impact. Distributed loading to the ribs due to airbags as well as rib and clavicle loading due to the shoulder belt make quasi-static thorax loading data also necessary. Performing such tests, the sternum of volunteers or cadavers is loaded with a plate with the subject’s back against a rigid structure. The applied load and the anterior-posterior deflection of the thorax are recorded. Reviewing the data available, Melvin et al. (1985) concluded that for deflections of up to 41 mm the thorax has an approximate linear stiffness of 26.3 N/mm, and for deflections greater than 76 mm, the stiffness increases to 120 N/mm. However, the results are influenced by the individual physique of the test subject and differ remarkably for different conditions of the test subject such as embalmed and unembalmed cadavers and relaxed and tensed volunteers. In Fig. 5.10 results obtained by Lobdell et al. (1973) are presented, clearly indicating the difference between a relaxed and a tensed volunteer. The fact that the stiffness of the thorax is increased in a tensed state can be regarded as beneficial in terms of injury tolerance.
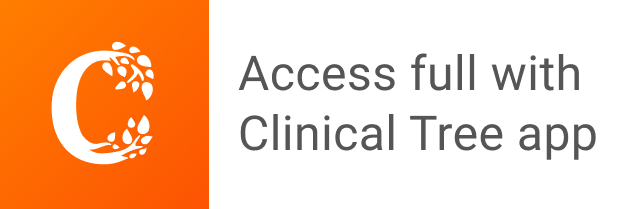