Fig. 28.1
Anatomy of the respiratory system

Fig. 28.2
Tracheobronchial tree anatomy
After the tracheal division into the right and left bronchus, there are subsequent divisions—total of 23 generations of divisions. The first 17 divisions carry gas but do not take part in gas exchange. Also, the respiratory mucosa gradually changes from ciliated columnar to cuboidal and finally to flat alveolar epithelium, where gas exchange takes place. Similarly, the airways gradually lose their cartilaginous support and finally their smooth muscle content.
Alveoli sacs begin to appear about the 17th generation/division. Each alveoli sac contains about 17 alveoli, which are thin walled and in close contact with the pulmonary capillaries. The alveoli are made up of Type I cells, which take part in gas exchange, and Type II cells, which produce surfactant. Type I cells form tight junctions preventing passage of large molecules, such as albumin, into the alveolus. Type II cells can divide and replace damaged Type I cells. The interstitial space contains mainly elastin and collagen.
Blood is supplied to the lungs by both the bronchial and the pulmonary arteries. The bronchial arteries arise from the aorta and provide nutritive needs of the airways. The pulmonary arteries arise from the right ventricle and form a capillary network, which takes part in gas exchange (O2 uptake and CO2 elimination). The pulmonary veins then supply oxygenated blood to the left atrium.
The tracheobronchial tree is innervated by both the parasympathetic (vagus nerve) and sympathetic nervous systems (beta-2 and alpha-1, primarily). Parasympathetic stimulation causes bronchoconstriction and increase in secretions, while sympathetic stimulation causes bronchodilation and decrease in secretions. The diaphragm is innervated by the phrenic nerves (C3–5).
Physiology
The lungs have various important physiological functions which are summarized in Table 28.1.
Table 28.1
Functions of the airway and lungs
Gas exchange |
Alter pH of blood |
Humidification and filtration of air |
Surfactant production |
Secretion of immunoglobulin-A to fight infections |
Secretion of mucus which has antimicrobial substances |
Metabolism of norepinephrine, serotonin, bradykinin, prostaglandins |
Synthesis of angiotensin-converting enzyme, which converts angiotensin I to active angiotensin II |
Lungs have high content of heparin and plasminogen activator—causing breakdown of fibrin debris |
Control of Breathing
Respiratory Centers
Breathing is controlled neuronally by respiratory centers located in the medulla and pons. The dorsal respiratory group in the medulla is responsible for inspiration, while the ventral respiratory group is responsible for expiration. While the medulla is responsible for the basic respiratory rhythm, the pontine centers seem to fine-tune the respiratory rate and rhythm. The lower pontine (apneustic) center is excitatory, while the upper pontine (pneumotaxic) center is inhibitory (Fig. 28.3).


Fig. 28.3
Neuronal control of breathing
Sensors
CO2 crosses the blood–brain barrier (BBB) and causes a decrease in cerebrospinal fluid (CSF) pH. The central chemoreceptors sense the change in pH, and compensate for the change by causing an increase in minute ventilation, thereby returning the CO2 back to normal (Fig. 28.4). Over the course of few days bicarbonate is transported across the BBB to return the pH of CSF to normal, which decreases the minute ventilation. It should be noted that while increasing PaCO2 tension leads to an increase in minute ventilation, very high PaCO2 tension (>80 mmHg) causes depression of respiration, which is called CO2 narcosis. Furthermore, under anesthesia the PaCO2 at which ventilation is Zero, that is, the highest arterial CO2 tension at which there is no spontaneous ventilation, is called the apneic threshold.


Fig. 28.4
Chemical control of breathing
Peripheral chemoreceptors, which are located in the carotid bodies and the aortic arch, also modulate respiration (Fig. 28.5). Stimulation of peripheral chemoreceptors (decreases in PaO2 or pH, or increases in PaCO2 levels) leads to an increase in minute ventilation. The peripheral chemoreceptors interact with the central respiratory centers via the glossopharyngeal nerves. Of the stimuli, the carotid bodies are most sensitive to changes in the PaO2 (especially below PaO2 < 50 mmHg), while the central chemoreceptors are most sensitive to changes in the PaCO2.


Fig. 28.5
Peripheral chemoreceptors
Other receptors that modulate respiration, though to a minor extent, are the stretch receptors located in the smooth muscle of airways, and irritant receptors located in the tracheobronchial mucosa. Stretch receptors prevent the lung from excessive expansion (inhibition of inspiration) and excessive deflation (shortening of expiration). Input from the stretch receptors is carried to the brain (centrally) by the vagus nerve. Irritant receptors produce bronchoconstriction or tachypnea in response to stimulation by smoke, dust, or light anesthesia.
Lung Compliance and Work of Breathing
The elastic properties of the chest wall and the lung make them move in opposite directions; the chest wall expands outwards, while the lungs recoil inwards. The chest wall and the lungs each has its own compliance, which is defined as the change in volume/change in pressure.




Total pulmonary compliance (lung and chest wall) is about 100 ml/cm H2O. Lung compliance is affected by lung volume, pulmonary blood flow, and pulmonary diseases. Lung compliance can be referred to be either static or dynamic. Static lung compliance is the change in volume for any given applied pressure, while dynamic lung compliance is the compliance of the lung at any given time during actual movement of air. The compliance of the lungs is different on inspiration and expiration for identical volumes, which is referred to as hysteresis. Compliance is greatest at moderate lung volumes, and much lower at volumes which are very low or very high.
In a normal awake patient, on inspiration of 500 ml of tidal volume, the transpulmonary pressure increases from 0 to 5 cm H2O (Fig. 28.6). Potential energy is stored during inspiration (active process), which is spent during expiration (passive process). Work of breathing can be calculated as:



Fig. 28.6
Work of breathing. Lung volume plotted against transpulmonary pressure. (a) Normal awake patient with lung compliance (slope of AB) of 100 ml/cm H2O, (b) Anesthetized patient with COPD with lung compliance (slope of AB) of 50 ml/cm H2O (I inspiration, E expiration). See text for more description

As shown in Fig. 28.6a, the work of breathing equals the area in the triangle ABC plus the shaded area. Triangle ABC represents the work to overcome elastic resistance, while the shaded area represents the work to overcome airflow resistance. In an anesthetized patient with COPD (Fig. 28.6b), increased work is required to overcome both the elastic resistance (triangle ABC) and the airflow resistance (shaded area). During expiration about half the air leaves passively, while the other half of the air has to be forced out. This requires energy, which increases the work of breathing. Therefore, an anesthetized patient with COPD has decreased lung compliance, increased elastic resistance (ABC), and increased airflow resistance during inspiration and expiration.
Airflow Resistance
High airway resistance leads to a decrease in lung volume (Fig. 28.7). The flow of gas in the lungs can be laminar or turbulent. A gas that is flowing laminar has the highest velocity in the center and decreased velocity in the periphery, which usually occurs in smaller airways. Turbulent flow occurs at high gas flows and is inversely proportional to the airway diameter. Turbulent gas flow usually occurs in larger airways, and is seen at branching (sharp angles) airway points. Airflow resistance is higher in the conducting medium-sized bronchi (Fig. 28.8). Larger bronchi have low airway resistance due to their larger caliber, while terminal smaller bronchi have low airway resistance because of their increased cross-sectional area.



Fig. 28.7
Inverse relationship between airway resistance and lung volume

Fig. 28.8
Airway resistance is highest in medium-sized bronchi, and decreases starting about the seventh generation
The Reynolds number can be used to predict laminar or turbulent flow. Reynolds number less than 1,000 predicts laminar flow, whereas a number greater than 1,500 predicts turbulent flow.


A gas, such as helium, which has a low density to viscosity ratio, will have a lower Reynolds number than air at the same velocity and therefore provide laminar flow. A helium–O2 gas mixture can be used to decrease turbulent flow and airway resistance to gas flow. Airflow resistance is usually not increased with the induction of general anesthesia, per se. However, conditions that do lead to an increase in airflow resistance under anesthesia include upper airway obstruction, laryngospasm, secretions or mucus plug, bronchospasm, use of small size ETTube, and obstruction of breathing apparatus (kinking of ETTube).
Lung Volumes and Flow-Volume Loops
Pulmonary function tests are used to diagnose, or monitor disease progression or treatment. Patients presenting for major surgeries, especially with existing lung disease, often undergo pulmonary function tests. However, it should be noted that PFTs are not routinely ordered for preoperative evaluation. Spirometry is commonly used to measure lung volumes and flows. Lung volumes and capacities are summarized in Fig. 28.9 and Table 28.2.


Fig. 28.9
Lung volume and capacities
Table 28.2
Lung volumes and capacities for a healthy 70 kg male
Volume/capacity | Definition | Value |
---|---|---|
Tidal volume (TV) | Volume of air inspired during normal breathing | 500 ml |
Inspiratory reserve volume (IRV) | Volume of air forcibly inhaled after a normal tidal volume breath | 3,000 ml |
Expiratory reserve volume (ERV) | Volume of air forcibly exhaled after expiration of normal tidal volume | 1,000 ml |
Residual volume (RV) | Volume of air remaining in the lungs after a forced exhalation (ERV) | 1,200 ml |
Functional residual capacity (FRC) | Volume of air remaining in the lungs after a normal expiration | 2,200 ml (ERV + RV) |
Inspiratory capacity (IC) | Maximum volume of air that can be inspired | 3,500 ml (TV + IRV) |
Vital capacity (VC) | Volume of air that can be fully expired after a full inspiration | 4,500 ml (TV + IRV + ERV) |
Total lung capacity (TLC) | Maximum volume of air in the lungs | 5,700 ml (TV + IRV + ERV + RV, or VC + RV) |
Functional Residual Capacity
This is the volume of air in the lungs at the end of a normal expiration. The FRC is directly proportional to height, greater in upright than supine position, is higher in males (10 %) than females, and is reduced in obese people (increased abdominal mass). Preoxygenating a patient with 100 % O2 increases their oxygen reserve, as the 21 % oxygen in air for the FRC (2,200 ml) is replaced with 100 % oxygen. As normal oxygen consumption is about 250 ml/min, more time is available if there is a problem of ventilating the patient on induction of general anesthesia. Induction of anesthesia reduces the FRC by about 15–20 % (400 ml). Many factors are responsible for this decrease in FRC, such as loss of muscle tone causing the diaphragm to shift cephalad, an increase in intrathoracic blood volume, a Trendelenburg (head down) position, and a change in thoracic cavity shape.
Closing Capacity (CC)
This is the volume at which the small airways begin to close. These airways lack cartilaginous support and need radial traction provided by the surrounding tissues for their patency. Higher CC means more intrapulmonary shunting as the small airways continue to be perfused but not ventilated. Therefore, people with higher CC are prone to hypoxemia. The CC increases with age, and is less than the FRC, but by the age of 45 years CC approximates FRC, and exceeds FRC by age 65 years.
Pulmonary function tests commonly measure the above lung volumes and capacities. Commonly used terms for PFTs include:
Forced vital capacity (FVC): volume of air that can be exhaled forcefully and quickly after maximal inhalation. Measuring forced vital capacity provides information of airway resistance
Forced expiratory volume (FEV): volume of air that can be exhaled with force in one breath. The volume of air can be measured at 1 s (FEV1—normal 3.2 L), 2 s (FEV2), or 3 s (FEV3)
FEV1/FVC ratio: normal is >75–80 %. In obstructive lung disease both the FEV1 and FVC are reduced, but the FEV1 is reduced to a greater extent than FVC, so the ratio is low. In restrictive lung disease both FEV1 and FVC are reduced proportionally, so the ratio may be normal.
Forced mid-expiratory flow 25–75 % (FEF25–75 %): Measurement of air flow halfway through an exhalation. Both FEV1 and FVC are effort dependent; however, FEF25–75 % is effort independent and may be a more reliable measurement of obstruction.
Peak expiratory flow (PEF): Measurement of how quickly one can exhale
Maximum voluntary ventilation (MVV): maximum volume of air that can be breathed in and out during 1 min
Diffusion capacity for carbon monoxide: normal is >80 %
Flow-Volume Loops
Flow-volume loops can be used to identify the presence of pulmonary disease (obstructive or restrictive), or airway obstruction. A flow-volume loop is generated (Fig. 28.10) by making the patient inhale deeply to TLC, then forcefully exhaling to empty the lungs to residual volume, and then quickly inhaling again to reach TLC. Flow (both expiratory and inspiratory) is plotted on the Y axis and the volume on the X axis. The normal expiratory portion of the flow–volume curve is characterized by a rapid rise to the peak flow rate, followed by a nearly linear fall in flow as the patient exhales toward residual volume. In contrast, the inspiratory curve is a relatively symmetrical, saddle-shaped curve. Changes in the contour of the loop can aid in the diagnosis and localization of airway diseases: obstructive, restrictive, or presence of tumors (Fig. 28.11).



Fig. 28.10
A typical flow-volume loop

Fig. 28.11
Flow-volume loops in various conditions
Physiologic Dead Space
During inspiration, a portion of the gas remains in the upper airway, where no gas exchange takes place. This space is called the anatomical dead space. The remainder of the gas is used as alveolar ventilation. However, all the alveoli do not take part in gas exchange, as they may not be perfused. This space is called alveolar dead space. The total dead space, that is, the sum of the anatomical and alveolar dead space, is called physiological dead space. Physiological dead space is approximately 1/3rd of the tidal volume, and is about 150 ml in adults or roughly 2 ml/kg. It can be calculated by the Bohr’s equation:


where PaCO2 is the arterial CO2 tension and ETCO2 is the expired CO2 tension. Factors affecting dead space are summarized in Table 28.3. ETCO2 is used as a measure of PaCO2, and the gradient between ETCO2 and PaCO2 is 5–7 mmHg (PaCO2 is higher, normal PaCO2 is 35–45 mmHg). This gradient is increased in diseased states and during some surgeries (laparoscopy).
Table 28.3
Factors affecting physiologic dead space
Increase | Decrease |
---|---|
Upright position | Supine position |
Age | Artificial airway |
Positive pressure ventilation | |
Anticholinergic drugs | |
Hypotension | |
COPD |
Alveolar Oxygen Tension
Inspired oxygen tension is reduced by water vapor in the breath in the upper airway. Therefore, alveolar oxygen tension is lower than the inspired oxygen tension. Alveolar gas is also diluted by residual alveolar gas from previous breaths and the addition of CO2. Alveolar gas oxygen tension can be calculated as:




where PiO2—inspired oxygen tension, PAO2—alveolar oxygen tension, PaCO2—arterial oxygen tension, RQ—respiratory quotient (normal 0.8) is the ratio of CO2 produced/O2 consumed, FiO2—delivered oxygen, PB—atmospheric pressure, PH2O—water vapor pressure). Furthermore, normal alveolar–arterial oxygen tension gradient (A-a gradient) is 15–20 mmHg. Normal PaO2 is 80–100 mmHg, and hypoxemia (PaO2 < 60 mmHg) results in an increased A-a gradient. Intrapulmonary shunting (no ventilation but perfusion) increases the gradient, while increasing mixed venous oxygen tension decreases the A-a gradient.
Hypoxic Pulmonary Constriction
Pulmonary hypoxia leads to vasoconstriction in the lung, called hypoxic pulmonary constriction (HPV). This effect is opposite to the systemic circulation. The end result is optimal perfusion in those areas of the lungs which are adequately ventilated. HPV leads to decreased shunting and preventing further hypoxemia.
HPV is an autoregulatory mechanism, which diverts blood flow from the atelectatic lung toward the remaining normoxic or hyperoxic ventilated lung. HPV maintains the PaO2 by decreasing the amount of shunt flow that can occur through hypoxic lung. HPV is of high importance when the percentage of hypoxic lung is intermediate (30–70 %), which is the case during single-lung ventilation. HPV is of little importance when very little of the lung is hypoxic (near 0 %) because the shunt will be small (normal 1–2 %), or when most of the lung is hypoxic (near 100 %), as there is no significant normoxic region to which the hypoxic region can divert blood flow. Factors which inhibit HPV are listed in Table 28.4.
Table 28.4
Factors inhibiting hypoxic pulmonary vasoconstriction
Very high or very low pulmonary artery pressures |
---|
Hypocapnia |
High or low mixed venous oxygen tension |
Pulmonary infections |
High airway pressures, high PEEP |
Low FiO2, or prolonged administration of high FiO2 (>50 %) |
Vasodilators—nitroprusside, nitroglycerin, dobutamine, calcium channel blockers, volatile inhalational agents >1.0 MAC |
Mixed Venous Oxygen Saturation
Blood is returned to the heart via the superior and inferior vena cava, and the coronary sinus. Blood then flows to the lungs from the right ventricle and into the pulmonary artery. Therefore, a blood sample obtained from a pulmonary artery catheter denotes a mixed venous oxygen sample. Venous blood has a PO2 of 40 mmHg and a Hb saturation of 75 % (60–80 %). MvO2 in various conditions is summarized in Table 28.5. MvO2 can be calculated by the following formula. Clinical relevance of continuous MvO2 monitoring includes use as a surveillance, early warning system, and to guide and adjust therapy.
(SaO2—arterial oxygen saturation, Hb is expressed in mg/L, hence a factor of 10, VO2—oxygen consumption per minute, CO—cardiac output)
Table 28.5
Mixed venous oxygen saturation in various conditions
High mixed venous oxygen saturation |
• Increased oxygen delivery |
– Increasing FiO2 |
• Decreased oxygen demand |
– Hypothermia, sepsis, left to right shunting, high cardiac output |
Low mixed venous oxygen saturation |
• Decreased oxygen delivery |
– Anemia, hemorrhage (low Hb) |
– Hypoxia (decreased arterial oxygen saturation) |
– Hypovolemia, shock, arrhythmias (decreased cardiac output) |
• Increased oxygen demand/consumption |
– Hyperthermia, pain, shivering |

Oxygen Transport
Approaching the topic of cardiopulmonary physiology from the concept of oxygen delivery is often most helpful. O2 delivery refers to the amount of oxygen that is transported or delivered to the tissues, and is expressed in milliliters of oxygen per minute. O2 delivery depends on two separate but interconnected concepts: First, the amount of oxygen that is actually carried in the blood, or the oxygen content, and second, the rate of blood (with associated oxygen) that is carried to the tissues (or cardiac output). Adult O2 stores are about 1,500 ml, and O2 consumption is about 250 ml/min. During anesthesia, it is imperative that O2 delivery and CO2 removal are maintained. This is frequently quite difficult to accomplish in patients with severe lung disease who are undergoing thoracic surgical procedures.
Blood oxygen content is based on two factors, the amount of oxygen bound to hemoglobin (Hb), and to a much lesser extent, the amount of dissolved oxygen. The basic equation for blood oxygen content is:


As can be seen from the equation, the primary determinant of oxygen content is the Hb-bound portion, which depends on the amount of hemoglobin and the oxygen saturation. Oxygen binds to Hb in a cooperative fashion, meaning as oxygen binds, it changes the conformation of the Hb molecule such that it facilitates further binding. This effect is seen in the “S” shape of the O2–Hb dissociation curve with its associated hysteresis. As the PO2 increases, oxygen binds more readily and the saturation, or % of oxygen bound to hemoglobin, rises rapidly until around 90 % where it begins to level off. The shape of the curve is altered or shifted under certain physiologic circumstances.
The curve is shifted to the left (meaning oxygen is more tightly bound to hemoglobin) under the conditions of alkalosis, decreased temperature, or decreased levels of 2,3 diphosphoglycerate (DPG)—a by-product of glycolysis. Carbon monoxide or cyanide poisoning and methemoglobinemia resulting from nitrates/sulfonamides also shift the curve to the left.
The curve is shifted to the right (meaning oxygen is less tightly bound at a given PO2) under the conditions of acidosis, hyperthermia, and increased 2,3 DPG (Fig. 28.12).
Fig. 28.12
Oxygen hemoglobin dissociation curve
An easy way to remember this is by recalling that tissues that are more metabolically active have increased temperature and CO2 (acid) production, and therefore need more oxygen delivered to them. Nature has provided for this by rightward shift of the O2–Hb dissociation curve with associated decreased affinity for oxygen and easier oxygen unloading to these active tissue beds.
Carbon Dioxide Transport
Approximately 80 % of CO2 transport occurs as bicarbonate ion, and rest 10 % each as dissolved and bound to amino groups on hemoglobin (carbamino-hemoglobin). Adults have about 120 L of CO2 stores. CO2 released from the red cell is acted on by the enzyme carbonic anhydrase (CA) to form carbonic acid (H2CO3), which dissociates into bicarbonate and hydrogen ions (Fig. 28.13).



Fig. 28.13
Carbon dioxide transport and chloride shift

Oxyhemoglobin (presence of oxygen) has a lower affinity to bind to CO2, and conversely deoxyhemoglobin (absence of oxygen) has a higher affinity to bind to CO2. In tissue beds, where O2 is consumed and its concentration is low, CO2 binds more readily to hemoglobin. Therefore, venous blood has more CO2 content than arterial blood. This property is known as the Haldane effect, and is responsible for increased CO2 transport by hemoglobin.
The reverse holds true in the lungs, where O2 levels are high and CO2 is preferentially off-loaded. Because CO2 is eliminated, the concentration of H+ is low in the lungs, which drives the binding of Hb to oxygen. The binding of Hb to oxygen further releases H+ ions, driving the formation and elimination of CO2. This property is known as the Bohr Effect, which refers to the inverse relationship between oxygen and hydrogen ion affinity for Hb. The two properties, the Haldane and Bohr effect, work in concert to increase O2 loading and CO2 off-loading in the lungs, and O2 release and CO2 binding in the tissues.
Another interesting property is the Chloride (Hamburger) shift. Bicarbonate is continually produced in the red cells. To prevent the red cell from becoming alkalotic, the bicarbonate ions are transported out and chloride ions are transported in. This maintains electrical neutrality, and is termed as chloride shift.
Ventilation–Perfusion
Effect of Position on Ventilation and Perfusion
In an upright position, the lower portions of the lungs are most ventilated and perfused, while in the supine position the dependent portions receive the highest ventilation and perfusion. Each lung can be divided into three zones (Fig. 28.14), which have a gradient between three pressures, the alveolar, the pulmonary arterial, and the pulmonary venous pressures. Zone 1 has the highest amount of dead space as the alveolar pressure is higher than the arterial/venous pressure, thereby occluding the pulmonary capillaries. Pulmonary capillary flow is intermittent in zone 2, while in zone 3 it is continuous.


Fig. 28.14
Zones of the lung showing the relationship between alveolar (PA), pulmonary artery (Pa), and pulmonary venous (Pv) pressures
Alveolar ventilation is on average 4 L/min, whereas perfusion is about 5 L/min. Therefore, normal V/Q ratio is 0.8. Most of the lung areas have a V/Q of 0.8–1.0, but V/Qs can range from 0.3 to 3.0. Theoretically, a V/Q of “0” means no ventilation, while a V/Q of “infinity” means no perfusion. The former is referred as a intrapulmonary shunt, while the latter is referred as alveolar dead space. Increase in the number of low V/Q units leads to hypoxemia, while an increase in high V/Q units leads to hypercarbia. The concentration of any gas in the alveolus is dependent on the rate of its addition (perfusion) and the rate of its removal (ventilation).
Nondependent areas of lung have higher V/Qs than do dependent areas of lungs, as the nondependent areas are ventilated better, while the dependent areas are perfused better. Shunt and dead space fall under the general heading of ventilation–perfusion (V/Q) mismatch. Essentially, there is a mismatch in the amount of pulmonary blood flow compared to the amount of alveolar ventilation within the lung. V/Q mismatch may occur globally throughout the lung or, more commonly, only in regional areas of the lung. In fact, it is quite possible to have areas of normal V/Q, areas of low V/Q (Q > V or shunt), and areas of high V/Q (V > Q, or dead space) all at the same time.
Venous Admixture (Low V/Q and Shunt)
Venous admixture refers to a process where pulmonary blood flow exceeds alveolar ventilation thus encompassing areas of lung with both low V/Q and shunt (V/Q = 0). A common clinical scenario causing pure shunt is pneumonia with alveolar flooding due to pus, debris, and/or fluid such that no ventilation takes place in the affected respiratory unit. Increased venous admixture due to right to left shunting occurs with single-lung ventilation in thoracic surgery. During the initiation of single-lung ventilation the patient experiences a fixed shunt of the non-ventilated side. Over time, the alveolar PO2 on the non-ventilated side falls, and hypoxic pulmonary vasoconstriction increases, thereby redirecting blood flow to the ventilated side with an associated reduction in the degree of shunt. Other etiologies of low V/Q are shown in Table 28.6.
Table 28.6
Etiology of high and low V/Q mismatch
High V/Q (Dead space) | Low V/Q (Shunt) |
---|---|
Pulmonary embolism | Chronic bronchitis, asthma |
Chronic obstructive pulmonary disease | Hepatopulmonary syndrome |
Shock-pulmonary vascular hypotension | Pulmonary edema |
Tumors | Pneumonia |
Pneumothorax | Significant atelectasis |
High airway pressures | Large pleural effusion, pneumothorax, hemothorax |
Acute respiratory distress syndrome |
The amount of shunt is reflected in the arterial oxygen partial pressure PaO2. In shunt physiology, the PaO2 falls significantly, whereas the PaCO2 remains normal to only slightly elevated. In mild/small shunt, increasing the FiO2 will increase the PaO2, but in a large shunt enriching the inspired oxygen up to 100 % will not reverse the low PaO2. The reason for this is based on the oxygen content of the blood. Even with hypoxic pulmonary vasoconstriction, portions of the lung that are perfused and ventilated can only take up oxygen to a certain extent. Once hemoglobin is fully saturated, adding more oxygen will only increase the dissolved O2 content by the factor of 0.003 × PaO2. This additional oxygen will not make up for the areas of lung that remain poorly ventilated with little or no gas exchange.
The reason the PaCO2 does not fall is more complicated. The CO2 content in the blood is kept much more constant than the oxygen content between the arterial and venous systems. The arterial PaCO2 is typically around 40 mmHg, whereas the venous PaCO2 is only around 45 mmHg. The PaCO2 has to be kept tightly controlled in order to keep blood pH relatively constant throughout the body. Therefore, there is simply not a huge difference between the PaCO2 in areas of the lung that have a low V/Q and those that are normal. Even with a very large shunt fraction, when the blood mixes in the pulmonary vein, the PaCO2 is only marginally elevated, and simply increasing the respiratory rate slightly will return the PaCO2 to a normal value.
High V/Q and Dead Space
High V/Q refers to ventilation–perfusion abnormalities which are characterized by areas of lung with high ventilation relative to perfusion (V/Q > 1), and impaired CO2 elimination. As discussed before, this is referred to as alveolar dead space. Pure dead space (opposite of shunt) are areas where ventilation infinitely exceeds pulmonary perfusion (V/Q = infinity). The classic example of increased dead space is a pulmonary embolism. In this case, the area of lung that is perfused by the blocked pulmonary artery receives no blood flow, and therefore no gas exchange takes place, and this is referred to as wasted/dead space ventilation. Other etiologies of high V/Q are shown in Table 28.6.
In dead space physiology, the PaCO2 rises dramatically, but the PaO2 does not fall nearly so far. The PaCO2 rises because there is significant wasted ventilation. The amount of change in the PaCO2 depends on the amount of dead space, as well as on the physiologic reserve of the individual. Patients will increase minute ventilation in an attempt to normalize their PaCO2, and will do so until they begin to fatigue, causing the minute ventilation to fall and the PaCO2 to rise further. The PaO2 level is maintained by blood flow being diverted to other areas of the lung that have a normal V/Q. In these areas Q will increase but so will ventilation in order to keep the PaCO2 constant. V/Q will be matched in these areas until the patient starts to fatigue, which will then cause the PaO2 to fall. The PaO2 can be normalized with the addition of supplemental oxygen.
Diseases of the Pulmonary System
Obstructive Airway Diseases
Obstructive airway diseases can be divided into two major forms: asthma and chronic obstructive pulmonary disease (COPD). These two diseases are fairly common, affecting millions of Americans, and are responsible for a large percentage of the US healthcare budget.
Asthma
Asthma is rapidly rising in prevalence. In 2011, it was estimated that 1 in 12 or 8 % of the general US population had asthma. This corresponds to approximately 25 million Americans, and is estimated to cost $56 billion dollars annually. Asthma does not affect the population equally. Children are more likely than adults to have asthma, and there is an increased prevalence in African Americans versus Caucasians or Hispanics. In fact, the prevalence is estimated to be 1 in 6 among black children.
Asthma, which is typified by chronic airway inflammation and hyperreactivity, causes a variable degree of airway obstruction, most seen on exhalation. Asthma has four major pathologic features:
Variable airflow obstruction (dyspnea, cough, wheezing)
Airway inflammation (edema, secretions)
Hyperresponsiveness of the airways to specific triggers such as cold, exercise, aspirin, and allergens (pollen, dust, pollutants, chemicals) may be seen in otherwise asymptomatic patients.
Reversibility (or at least partial reversibility) of airway obstruction with inhaled bronchodilators
On exposure to a trigger, typically two processes happen: a parasympathetic (vagal response), and release of chemical triggers, such as histamine, bradykinin, prostaglandins (PGE2/F2α/D), and leukotrienes, which all lead to bronchoconstriction. Bronchoconstriction combined with mucosal edema and secretions leads to an increase in airflow resistance.
Signs and Diagnosis
Patients with asthma typically present with cough, shortness of breath, and/or wheezing. However, these symptoms may be quite variable, as the degree of inflammation, airflow obstruction, or hyperresponsiveness is unique in each individual. Prolonged or severe asthmatic attacks markedly increase the work of breathing and lead to muscle fatigue and shunting with increase in the number of areas of low V/Q ratios. Hypoxemia leads to tachypnea, which drives down the PaCO2. Therefore, a patient having an asthmatic attack, with a normal or elevated PaCO2, is often a sign of impending respiratory failure. Severe respiratory obstruction may also be associated with ST-segment changes and heart strain.
Typically, asthma begins early in life for most patients and is characterized by risk factors such as atopy, recurrent wheezing, or a parental history of asthma. The initial diagnosis of asthma is most typically made by the presence of expiratory wheezing on physical exam. Confirmation of this diagnosis is usually done by spirometry demonstrating airflow obstruction, and also by bronchoprovocation of airway hyper-responsiveness with methacholine and bronchodilation with inhaled albuterol.
There is a subgroup of approximately 5–10 % of asthmatics who have severe or refractory asthma as defined by the American Thoracic Society (ATS). Despite the small percentage of severe asthmatics, these patients experience most of the significant morbidity associated with this disease. The ATS has established major and minor criteria for the diagnosis of severe asthma. A patient can be classified as having severe or refractory asthma if they demonstrate at least one major and two of the minor criteria.
The major criteria for severe or refractory asthma are the continuous (or near continuous) use of oral corticosteroids, or needing high-dose inhaled corticosteroids
The minor criteria include the need for daily treatment with a bronchodilator, persistent airway obstruction (FEV1 less than 80 % predicted), or peak expiratory flow variability greater than 20 %
Additional minor criteria include the need for urgent care visits for asthma, use of 3 or more steroid bursts in a year, increase in corticosteroid use, prompt deterioration in function, or a near fatal asthma event in the past
Pulmonary function studies and peak expiratory flow measurements demonstrate evidence of airflow obstruction and are the mainstay of diagnosis and monitoring of therapy. Besides reduction in FEV, there is an increase in FRC, RV, and TLC. Reversibility of obstruction after administration of a bronchodilator helps to confirm a diagnosis of asthma. An increase in FEV1 % predicted of more than 12 % and greater than 0.2 L has historically been used as the criteria for bronchodilator responsiveness and variability of airflow obstruction. However, current data suggest that this may not be the “gold standard” criteria, since in a recent study only slightly more than 50 % of asthmatics met this standard. Therefore, a more holistic approach is needed to make an accurate diagnosis of asthma. This should include a combination of patient history, symptoms, and pulmonary functions tests on a case by case basis.
Treatment
The National Heart Lung and Blood Institute (NHLBI) has set guidelines for the effective treatment of asthma. This treatment has been divided into four components of care: Assessment and monitoring, education, controlling environmental factors and comorbid conditions, and finally, pharmacologic therapy. Additionally, the NHLBI has divided pharmacologic therapy into three distinct age groups: 0–4 years of age, 5–11 years of age, and those patients 12 years and older. The actual details of treatment for each of these groups are beyond the scope of this chapter, but in general the goal of pharmacotherapy is along a stepwise approach.
Initially, inhaled corticosteroids (beclomethasone, triamcinolone) should be initiated for effective long-term control (maintenance therapy) of asthma symptoms. If symptoms persist, then bronchodilator therapy with a β2-selective agonist (albuterol) is added to the treatment regimen. These agents stimulate the receptors to increase the activity of the enzyme adenylate cyclase, which leads to an increase in the concentration of cAMP. Additionally, patients may also be treated with ipratropium, an anticholinergic drug acting on muscarinic receptors.
During severe asthma exacerbations, intravenous therapy with glucocorticoids (hydrocortisone, methylprednisolone, followed by oral prednisone) is the mainstay of therapy. It should be remembered that glucocorticoids take about 4–6 h to take effect. In rare circumstances, when life-threatening status asthmaticus persists despite aggressive pharmacologic therapy, it may be necessary to consider general anesthesia (isoflurane or sevoflurane) in an attempt to produce bronchodilation. However, giving an inhaled anesthetic agent can be problematic if a patient is in severe status asthmaticus, since their respiratory function may be so compromised that drug delivery becomes unreliable. When general anesthesia is needed during severe asthmatic exacerbations, intravenous ketamine maybe an effective approach because of its sympathomimetic action and relaxation of increased airway smooth muscle tone. However, ketamine markedly increases secretions which may limit its usefulness in this situation.
Anesthetic Considerations
Preoperative Preparation
Thorough history and physical examination, with inquiry about any emergency room visits or hospitalization for asthmatic attacks, and steroid therapy. Absence of wheezing should be noted by chest auscultation. A chest radiograph may be useful for assessing air trapping (hyperinflation leading to hyperlucent lung fields).
Pulmonary function studies (normal values-FEV1>3 L for men and 2 L for women, FEV1/FVC> 75 %, PEFR>200 L/min) obtained before and after bronchodilator therapy may be indicated in the asthmatic patient who is scheduled for a thoracic or abdominal operation.
Measurement of arterial blood gases before proceeding with elective surgery is a consideration if there are questions about the adequacy of ventilation or arterial oxygenation. Hypocapnia is indicative of moderate disease, while hypercapnia is indicative of severe disease.Full access? Get Clinical Tree
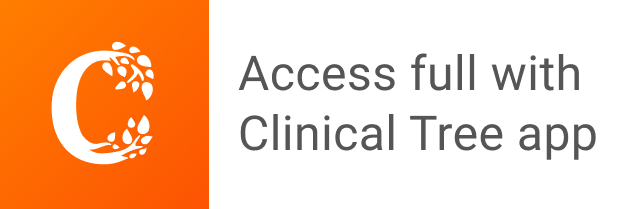