Fig. 8.1
Spinal cord central pattern generator (CPG) components. The point of control is the level of excitability of segmental interneurons, which is determined by the integrated activity of the other components. Disturbance of any component results in CPG uncoupling which is detected by reflexes and F-responses from the output through anterior horn cells
Spinal Cord Pathophysiology
Acute spinal cord transection causes spinal shock that is characterized by complete paralysis, hyporeflexia, loss of sensation, and muscle hypotonia caudal to the lesion. Spinal cord injury (SCI) disrupts or disinhibits the suprasegmental influence over segmental interneurons mediating presynaptic inhibition. The hyporeflexia associated with spinal shock may be due to an increase in the efficacy of presynaptic inhibition [12]. Over time, presynaptic activity decreases, resulting in enhanced spinal reflexes [13]. Observations in cats indicate that rostral acute SCI causes postsynaptic caudal lumbar motor neuron changes. In cats, rostral acute SCI causes hyperpolarization of caudal lumbar segment motor neurons [14–17]. Immediately following rostral spinal cord transection, the monosynaptic reflexes from the medial and lateral gastrocnemius, soleus, posterior biceps, and semitendinous muscles are reduced in amplitude or completely absent. During the 6 h following transection there is some recovery of reflex activity [18]. When using cold as a model for acute reversible spinal cord transection, hyperpolarization of caudal motor neurons occurs within 30 s and monosynaptic reflex amplitudes are gradually decreased. These changes persist during the application of cold. Rewarming restores reflex amplitudes to original values in 30 s, and the resting motor neuron membrane potential back to the original value in 1 min [14–16].
The hyperpolarization of motor neurons following spinal cord transection or cooling is thought to be secondary to decreased suprasegmental facilitation of motor neurons, which usually keeps them in a slightly hypopolarized state [14–17]. Fusimotor drive is also depressed early after acute spinal cord injury, for gamma motor neurons are also hyperpolarized [19]. H-reflexes and F-responses reflect the level of excitation of a large percentage of the motor neuron pool in the spinal cord gray matter [20, 21]. These recordings may be very helpful in detecting intraoperative spinal cord ischemic insults, since the level of gray matter excitability is depressed more with ischemia than is posterior column function. In animals the dorsal horn potential that is generated by postsynaptic gray matter activity disappears within 3–5 min after cessation of spinal cord perfusion. Posterior column potentials persist for 12–15 min [22].
Changes in spinal cord electrophysiologic signal processing can be used to help understand the electrophysiologic mechanisms occurring during acute SCI [23]. Changes in serial, parallel, and oscillatory processing, hyperpolarization, inhibition, and disinhibition may be observed.
Spinal Nerve Root Pathophysiology
Spinal nerve roots are more susceptible to injury than are peripheral nerves [24]. Spinal nerve roots are susceptible to injury by two mechanisms. The first is that proximally the dorsal and ventral roots split into rootlets and minirootlets [25]. The area where this split occurs is the central-peripheral transitional region, which is the point where the nerve root is more susceptible to mechanical injury. The axons at this point are enclosed by a thin root sheath, cerebrospinal fluid and meninges, and lack the protective covering of epineurium and perineurium that is present in peripheral nerve [26]. The second mechanism of injury is that there is an area of hypovascularity at the junction of the proximal and middle one-third of the dorsal and ventral roots. This is the point of anastomosis between the central vasa corona and peripheral segmental vessels. At this point, the nerve roots are more susceptible to mechanical injury [25].
The relationship between compression and traction forces on nerve conduction is well described in peripheral nerves and nerve roots [24, 27, 28]. The conduction abnormalities are coupled to the perfusion of the nerve root [24, 28]. A 3-mm retraction distance (pressure around 70 g/cm2) caused the intraneural blood flow to decrease to 20 % of initial value [28]. If nerve root dysfunction is recognized in the reversible phase, permanent injury can be avoided. Compression and traction may produce a mechanical effect on the nerve tissue in addition to compromising the blood supply to the nerve. Physiologic block is the first sign of nerve dysfunction and can be reversed in seconds.
When more axons in the nerve are blocked, the latency of the averaged nerve action potentials may increase and the amplitude may decrease. A more intense or prolonged compression or traction may produce myelin deformation at the edges of compression and prolongation of the nerve action potential latencies [28]. Further mechanical loading may produce a conduction block from segmental demyelination with reductions in action potential amplitudes. With injury, reversible functional changes precede more severe morphologic nerve changes such as neurapraxia (segmental demyelination) and axonotmesis (Wallerian degeneration). Although both these morphologic changes may be reversible in weeks to months, perineural and intraneural scarring may result and propagate further nerve damage [26, 27, 29, 30].
The mechanosensitivity of normal and abnormal dorsal root ganglia and axons has been defined in human and animal models [24]. In a cat model, rapidly applied mechanical forces induce short-duration (200 ms) impulses in normal nerve root. Static mechanical forces do not induce impulses in normal nerve root. Rapidly and statically applied mechanical forces induce long periods (15–30 s) of repetitive impulses in irritated or in regenerating nerves in continuity. Minimal acute compression of normal dorsal root ganglion induces prolonged (5–25 min) repetitive firing of nerve [31]. Mechanically induced trains of nerve action potentials can desynchronize afferent 1a nerve action potentials during H-reflex recordings and decrease the H-reflex amplitude. When interpreting intraoperative motor nerve root studies, it is important to understand the pathophysiologic mechanisms of nerve root injury and to understand the response of normal and pathologic nerve to not only different types of mechanical force, but also to electrical stimulation.
Late Responses
Following electrical stimulation of a mixed sensory–motor peripheral nerve, the compound motor action potential (CMAP) or M-wave is recorded from the peripherally innervated muscle. The M-wave is the result of orthodromic motor conduction from the point of stimulation to the muscle. In addition to the short latency M-wave, three late responses can be recorded: H-reflex, F-response, and A-wave (axon reflex). The mechanisms responsible for generating F-responses and A-waves are different from H-reflexes [32]. When recording intraoperative H-reflexes, the F-response and A-wave should not be confused with H-reflexes.
The F-response is not a reflex. After supramaximal stimulation of a mixed peripheral nerve, antidromic motor nerve impulses are conducted proximally to the ventral horn where they activate from 1 to 5 % of the motor neurons. This is followed by orthodromic conduction through motor fibers, and the F-response is recorded from muscle. The F-response is of low amplitude, and the latency, configuration, and duration vary from one stimulus to the next. This variability occurs because each time the motor neuron pool is activated, a different population of 1–5 % of the motor neuron pool is activated that have different conduction characteristics [33]. Both afferent and efferent components of the F-response follow the same motor neurons. The subpopulation of the motor neuron pool activated by the F-response and H-reflex are not the same [34]. F-response persistence is a measure of the excitability of the motor neuron pool. Persistence is the number of recorded F-responses divided by the number of stimuli. Clinically, a persistence less than 50 % is considered to be abnormal [35] (Fig. 8.2).
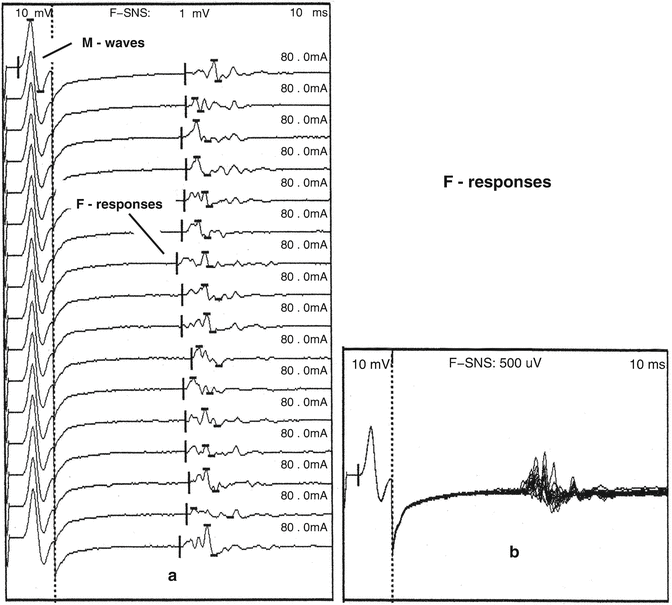
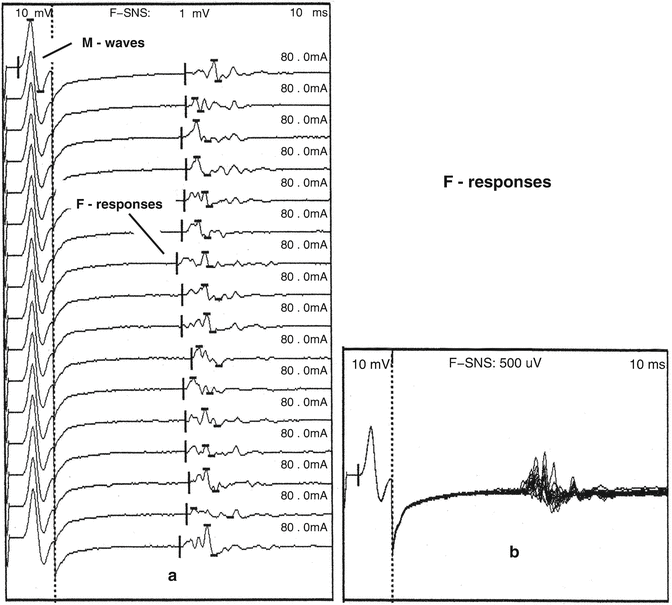
Fig. 8.2
F-responses are generated by antidromic motor impulses that activate 1–5 % of the motor neuron pool. The amplitude, latency, duration, and configuration change with each stimulus. Sequentially, recorded abductor hallucis F-responses (a) following tibial nerve stimulation at the ankle that are superimposed in (b)
The A-wave is a late motor response that is present with constant latency, configuration, and amplitude. Low-intensity stimulation elicits the A-wave, and it is usually blocked by higher intensity of stimulation. A-wave latency is between the CMAP and the F-response latency or exceeds the F-response latency. It may also appear with a latency between the M-wave and H-reflex latency or the latency may exceed the H-reflex latency. A-wave amplitude is less than the H-reflex amplitude. The A-wave should not be confused with the H-reflex or F-response. The A-wave is generated by peripheral nerve changes rather than changes in the central nervous system signal processing. The physiology of the A-wave is that there is peripheral neural damage with the presence of a collateral sprout from a proximal point of damaged motor nerve. The collateral sprout innervates muscle. When the antidromic impulse reaches the point of damage, a portion of the electrical impulse proceeds distally along the collateral sprout, and a small portion of the muscle is activated. Depending on the nerve stimulated, A-waves may be normal or abnormal (Fig. 8.3) [32].
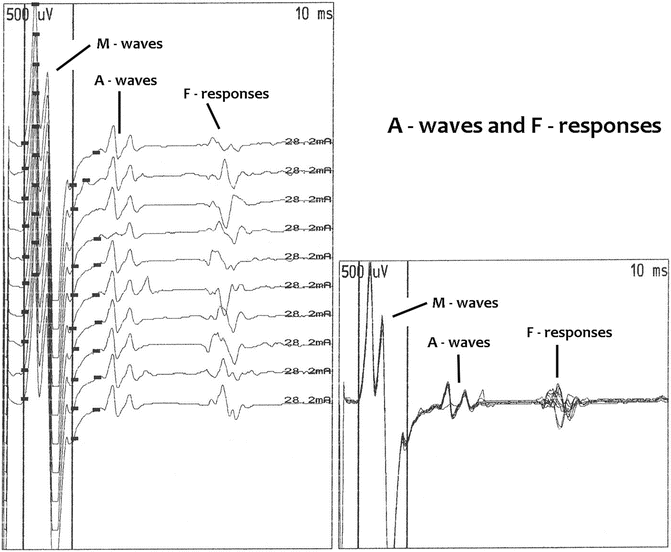
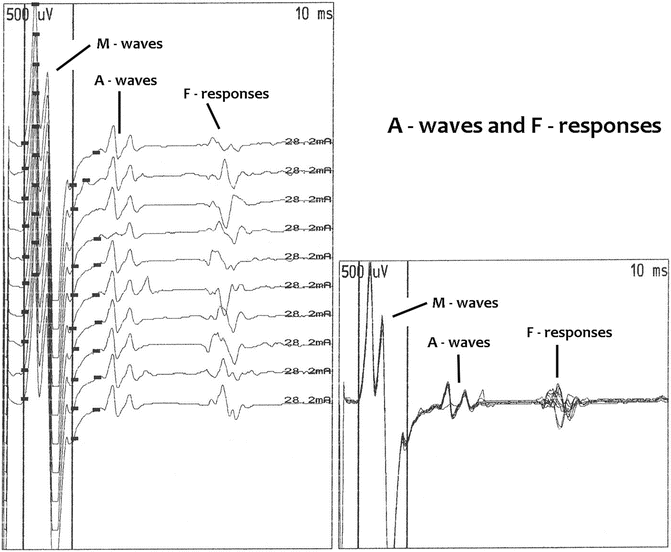
Fig. 8.3
A-wave amplitude, duration, latency, and configuration do not change from one stimulus to the next, but F-responses do. These are ten abductor hallucis recordings following tibial nerve stimulation at the ankle
H-Reflexes: Monosynaptic, Oligosynaptic
See Chap. 7 for a discussion of H-reflex testing.
Neurophysiologic Basis of H-Reflexes
The H-reflex is a CMAP recorded from muscle after electrical afferent activation of a monosynaptic reflex. The afferent pathway involves electrical activation of the large 1a nerve fibers originating from muscle. After entering the dorsal horn of the spinal cord, the 1a fibers synapse with the motor neurons. The efferent pathway involves orthodromic motor conduction through motor fibers in the same homologous spinal segment as the afferent pathway [32]. In normal newborns, H-reflexes may be recorded from many widely distributed muscles. After 2 years of age, they are primarily present in the gastrocnemius, soleus, and flexor carpi radialis muscles. The more restricted distribution of H-reflexes in adults reflects the refinement of motor neuron pool activation with central nervous system maturation. In adults, they are also frequently found in the quadriceps and plantar foot muscles [36–38].
The H-reflex was first described by Hoffman in 1918 [39] and characterized more in the 1950s [40]. The reflex is most easily recorded from the gastrocnemius muscle after stimulation of the tibial nerve in the popliteal fossa. The fast-conducting low-threshold 1a fibers are activated with long-duration (1 ms) low-intensity stimulation. The stimulation rate is 0.5 Hz. The intensity of stimulation is slowly increased. Low-intensity stimulation activates the 1a fibers before the motor fibers, so at low intensity of stimulation, the H-reflex appears before the M-wave. The H-reflex CMAP is usually of a biphasic or triphasic configuration. The 1a fibers are activated first either because they have a lower threshold than motor fibers to long-duration stimulation or because they are located anatomically more superficial than the motor fibers in the popliteal fossa [41].
As the intensity of stimulation is increased, a greater percentage of the motor neuron pool is activated and the H-reflex amplitude increases [32]. In an awake human, when recording the gastrocnemius H-reflex, the percentage of the motor neuron pool activated averaged 50 % (range, 24.0–100 %) [20]. The motor neurons recruited with an increasing intensity of stimulation obey the Henneman size principle . Low-force motor neurons are recruited with low intensity of stimulation, and high-force motor neurons are recruited with higher intensity of stimulation [42].
The H-reflex amplitude usually peaks at or just before the M wave becomes present. Further increases in stimulation intensity result in a steady increase in the M-wave amplitude. When the M wave no longer increases in amplitude, the H-reflex is usually replaced by the F-response (Fig. 8.4). When the stimulus intensity is held constant from one stimulus to the next, the H-reflex is of short latency, short duration, simple configuration, and constant amplitude [32]. To determine if a CMAP is a H-reflex, the amplitude should exceed the M-wave amplitude, and the configuration and latency should be the same from one stimulus to the next [32]. Changes in the effect of the central facilitation and inhibition on the spinal interneurons and motor neurons may change the pattern of how the H-reflex is activated. With an increased presynaptic inhibition and hyperpolarization of motor neurons, the M-wave may be present before the H-reflex, and the H-reflex amplitude may be smaller than the M-wave amplitude. With a decreased presynaptic inhibition, the H-reflex may be robust, and it may not be possible to suppress and replace it with the F-response.
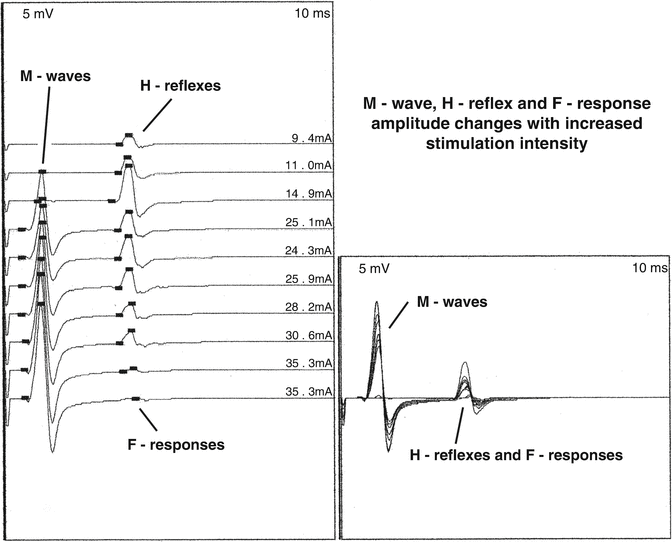
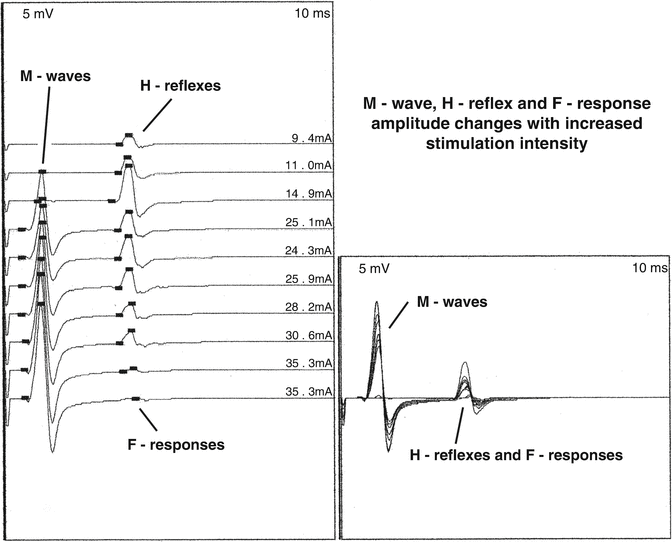
Fig. 8.4
The H-reflex is the first component elicited from the gastrocnemius muscle with increasing stimulus intensity of the tibial nerve in the popliteal fossa. The second is the M-wave and the third is the F-response
The H-reflex has been thought of as a monosynaptic reflex . The central conduction time between dorsal and ventral roots reveals only enough time for one synapse, that is, between 0.5 and 1.0 ms [32]. There is also evidence to indicate that the H-reflex is an oligosynaptic reflex. Low-threshold motor neurons may be activated by a single (monosynaptic) synapse through the fastest 1a afferents. Higher threshold motor neurons may be activated through several (oligosynaptic) synapses by the fastest 1a afferents and through single synapses by slower 1a afferents [32].
In the lower extremity of man, there are two types of prewired monosynaptic 1a H-reflex connections: homonymous (homosynaptic) and heteronymous (heterosynaptic). They are both part of the functional synergistic spinal cord CPGs. Both types may be recorded in the operating room. Homonymous monosynaptic H-reflexes are H-reflexes that are recorded from muscles that are innervated by the same nerve root as the 1a-activated sensory fibers. The gastrocnemius H-reflex is an example of a homonymous monosynaptic H-reflex (Fig. 8.5). 1a sensory action potentials may also make monosynaptic connections with motor neurons at spinal cord levels other than the 1a sensory segmental level. As a result of this activation, H-reflexes may be recorded from muscles having segmental innervation other than the 1a segmental afferent activation. These H-reflexes are called heteronymous monosynaptic H-reflexes (Fig. 8.6).
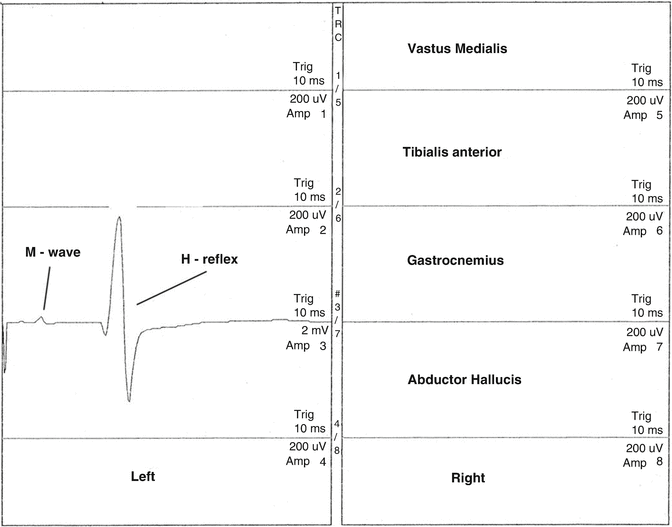
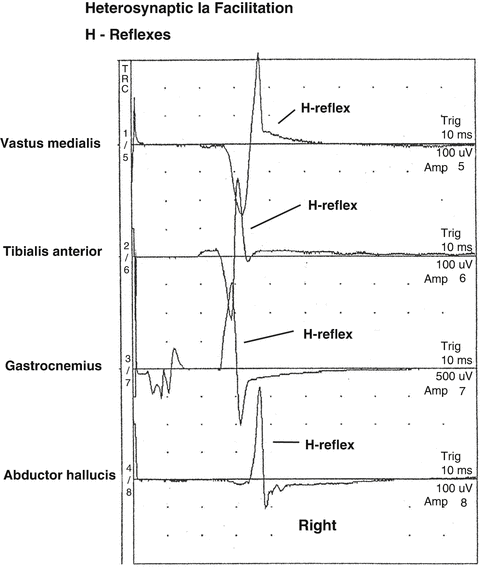
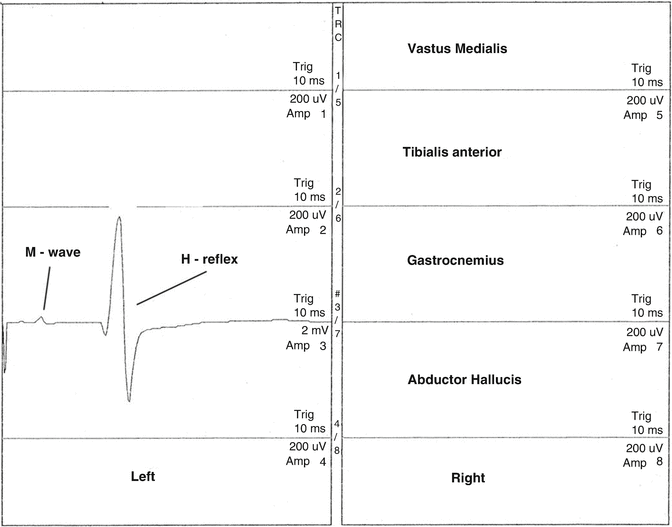
Fig. 8.5
Homonymous monosynaptic left intraoperative gastrocnemius H-reflex recorded following electrical stimulation of the left tibial nerve in the popliteal fossa
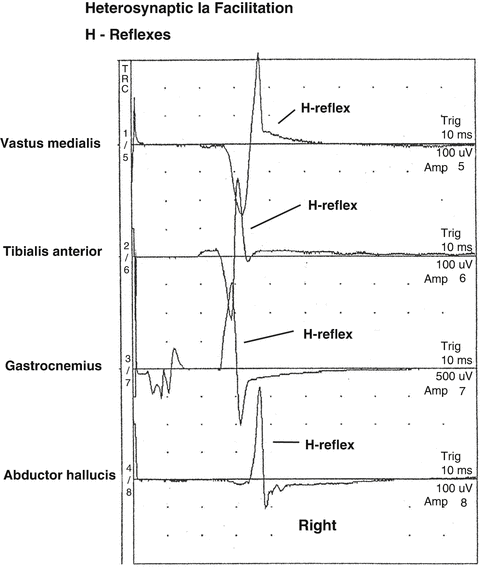
Fig. 8.6
Following intraoperative low-intensity electrical stimulation in the right popliteal fossa, decreased spinal cord presynaptic inhibition may allow not only the homonymous H-reflex to be recorded from the gastrocnemius muscle but also heteronymous H-reflexes may be recorded from the vastus medialis, tibialis anterior, and abductor halluces muscles
In humans, heteronymous connections normally exist between ankle and knee muscles. Functionally heteronymous connections provide coupling between muscles operating at different joints. These transjoint monosynaptic connections are important for maintaining equilibrium during bipedal stance and during gait. Intraoperatively, heteronymous H-reflexes are inhibited by presynaptic inhibition. When presynaptic inhibition is decreased, heteronymous H-reflexes may become present [43].
The presence of H-reflexes in muscles where they are not usually recorded can be an indication of a lesion of the suprasegmental central nervous system that results in a decreased effect of presynaptic inhibition on motor neurons. The abnormal distribution of H-reflexes in adults, such as in the tibialis anterior and intrinsic hand muscles, may indicate a disordered central motor system state. These changes occur because of uncoupling of the different components of CPGs [44–47].
Gastrocnemius H-Reflex
Gastrocnemius H-Reflex Normal Parameters
In the lower extremity , the H-reflex can be recorded from the gastrocnemius and soleus muscle after electrical stimulation of the posterior tibial nerve in the popliteal fossa [48]. This reflex is mediated by segmental S1 afferent and efferent activity [45]. Intraoperative normal parameters have not been established. Normal parameters established for clinical studies may serve as a guide for intraoperative studies. Clinically, the gastrocnemius H-reflex latency varies with age and leg length and has a mean latency of 28.9 ± 2.7 ms in an awake human. A regression equation may be used to calculate the expected latency for each individual: H-reflex (in ms) = 9.14 + 0.46 (leg length in centimeters) + 0.1 (age in years). A normogram based on this equation is available as a reference [49]. Clinically, the normal side-to-side amplitude difference between 21 and 67 years of age may reach 60 % [50]. The upper limit of normal clinical side-to-side latency difference is 1.5 ms [37]. When measuring latency, the most concise departure from the baseline occurs when the active recording electrode is over the motor point. It is therefore necessary to know the location of the motor point of the gastrocnemius muscle [51]. The H:M ratio is a measure of H-reflex motor neuron pool activation or excitation. It is calculated by dividing the maximum H-reflex amplitude by the maximum M-wave amplitude. It is normally less than 0.7 [37]. Intraoperatively, onset latencies may be greater because of the decreased limb temperature. In the operating room, H-reflex and M-wave amplitudes that are recorded in the clinical setting may be reduced by the use of neuromuscular junction (NMJ) blocking agents. Intraoperatively, the gastrocnemius H-reflex parameters that have been monitored are H-reflex amplitude, latency, and the H:M ratio. Right–left amplitude and latency differences are also used.
Gastrocnemius H-Reflex Stimulation and Recording Techniques
Stimulation is with needle or surface electrodes . The cathode is placed proximally in the popliteal fossa between the tendons of the medial and lateral hamstring muscles. The anode is placed 2–4 cm distal to the cathode. The stimulation rate is 0.5 Hz and the stimulus duration is 1.0 ms. The stimulus intensity is adjusted so that the H-reflex amplitude is maximal. The most effective stimulus intensity is chosen such that any increase or decrease in stimulus intensity results in a decrease in the H-reflex amplitude. Baseline recordings are made with the patient anesthetized before the start of the surgical procedure. Any variability in latency and amplitude should be noted in the baseline recordings.
For subdermal electroencephalographic (EEG) recording, needle electrodes are inserted in the medial head of the gastrocnemius muscle. The H-reflex may also be recorded in the calf from the soleus muscle. The technique for recording the H-reflex from the soleus muscle is the same as that for recording from the gastrocnemius muscle, only that the recording electrodes are placed over the mid-dorsal line of the leg with the active electrode 4 cm above the point where the two heads of the gastrocnemius muscle join the Achilles tendon. The reference electrode is placed 3 cm distal to the active electrode [48]. Monopolar electromyographic (EMG) needle electrodes and longer uncoated stainless steel needle electrodes may also be used.
Fine Teflon-coated silver wires with the wire exposed at the end and that are inserted with a spinal tap needle may also be used for recordings when the subcutaneous tissue is thick. The active electrode is inserted at the motor point of the gastrocnemius muscle and the reference electrode is inserted over tendon or bone. The needles are secured with tape. A range of different high- and low-frequency filters are used. A high-frequency filter of 10 KHz and a low-frequency filter of 20 Hz are most often used [32]. The time base is 100 ms. Recordings are single sweep.
In addition to recording H-reflexes from the gastrocnemius muscle , recordings may also be made bilaterally and simultaneously from the vastus medialis, tibialis anterior, and abductor hallucis muscles. Recording from these muscles will allow for the detection of heteronymous H-reflexes. This also allows for the monitoring of proximal vestibulospinal and reticulospinal controlled motor neurons and distal rubrospinal and corticospinal controlled motor neurons [11]. The gastrocnemius H-reflex may be used to monitor peripheral tibial nerve, proximal sciatic nerve, sensory and motor S1 nerve root, and S1 segmental spinal cord function. These reflexes can also be used to monitor the function of a variety of suprasegmental descending spinal cord systems that control the S1 segmental interneurons [52]. When present, heterosynaptic H-reflexes may be used to monitor other nerve roots. The ability to record lower extremity H-reflexes may be affected by pre-existing abnormalities, such as a generalized polyneuropathy, plexopathy, or radiculopathy. H-reflexes may be absent, latencies may be prolonged, amplitudes may be decreased, and the CMAP configuration may change. Amplitudes may also be decreased with the presence of a myopathy [1, 2].
Flexor Carpi Radialis H-Reflex
Flexor Carpi Radialis H-Reflex Background and Normal Parameters
In the upper extremity, the flexor carpi radialis H-reflex can be recorded after electrical stimulation of the median nerve over the distal medial upper arm or over the anterior medial elbow. This reflex is mediated by segmental C6/C7 afferent and efferent activity (Fig. 8.7). Intraoperative normal parameters have not been established. Normal parameters established for clinical studies may serve as a guide for intraoperative studies. Clinically, the flexor carpi radialis H-reflex latency varies with the arm length. When measuring latency, the most concise departure from the baseline occurs when the active recording electrode is over the motor point. It is therefore necessary to know the location of the motor point of the flexor carpi radialis muscle [51]. In awake humans, the mean latency is 17.07 ± 1.77 ms. The interlatency time is calculated by subtracting the M wave from the H-reflex latency. The interlatency time mean latency is 14.5 ± 1.8 ms. The maximum side-to-side H-reflex latency difference is 0.002 ± 0.42 ms. The maximum side-to-side interlatency time latency is 0.11 ± 0.44 ms. A regression equation is used to calculate the expected H-reflex latency: H-reflex (in ms) = 0.29 ± 0.195 × arm-length in centimeters. The equation for the interlatency time is −2.08 + 0.1878 × arm-length in centimeters. A normogram based on these equations is available as a reference. The arm length is measured from the tip of the third finger to the C6 spinous process with the arm pronated and the shoulder abducted to 90° [53, 54]. Intraoperatively, onset latencies may be greater because of the decreased limb temperature. Flexor carpi radialis H-reflex parameters that have been monitored are H-reflex amplitude, latency, and the H:M ratio. Right–left latency and amplitude differences are also used.
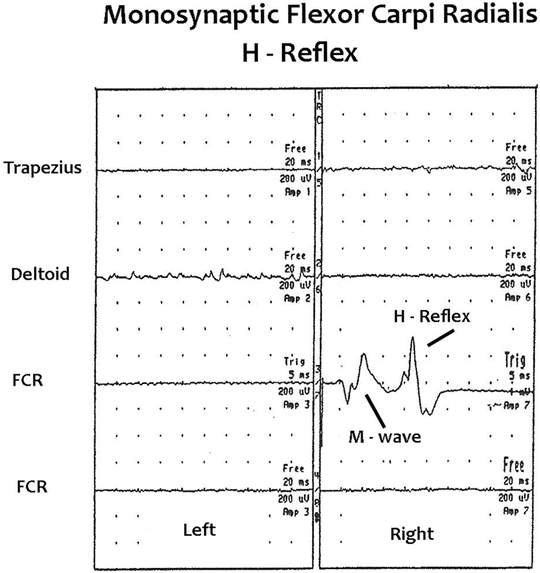
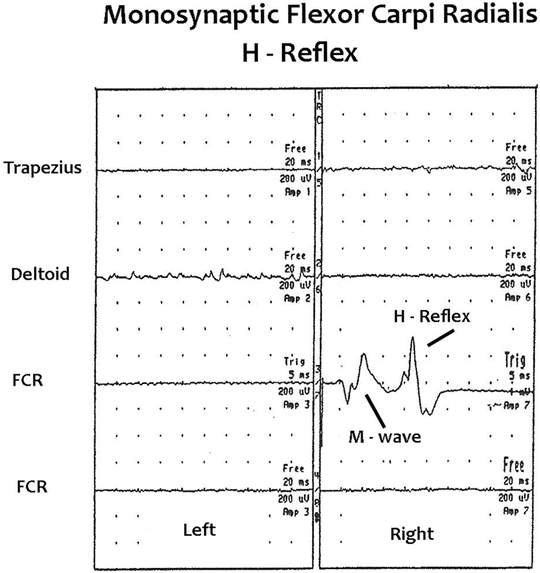
Fig. 8.7
Baseline intraoperative right flexor carpi radialis M-wave and H-reflex recordings. Free-run EMG activity is also recorded bilaterally from the trapezius, deltoid, and flexor carpi radialis muscles. Baseline free-run EMG activity is present in the left deltoid muscle secondary to left C5 and/or C6 nerve root irritation
Flexor Carpi Radialis H-Reflex Stimulation and Recording Techniques
Stimulation is with needle electrodes spaced 2 cm apart unilaterally over the distal medial upper arm or over the anterior medial elbow at 0.5 Hz and 1.0 ms duration. The cathode is proximal. The stimulus intensity is adjusted so that the H-reflex amplitude is maximal. The most effective stimulus intensity is chosen such that any increase or decrease in stimulus intensity results in a decrease in the H-reflex amplitude. Baseline recordings are made with the patient anesthetized before the start of the surgical procedure. In the operating room, H-reflex amplitudes that are recorded in the clinical setting may be reduced by the use of NMJ-blocking agents. Any variability in latency and amplitude should be noted in the baseline recordings.
For recording subdermal EEG , needle electrodes are inserted in the flexor carpi radialis muscles. Monopolar EMG needle electrodes and longer uncoated stainless steel needle electrodes may also be used. Fine Teflon-coated silver wires with the wire exposed at the end that are inserted with a spinal tap needle may also be used for recordings when the subcutaneous tissue is thick. The active electrode is inserted at the motor point and the reference electrode is inserted distally over tendon or bone. The needles are secured with tape. A range of different high- and low-frequency filters are used. A high-frequency filter of 10 KHz and a low frequency filter of 20 Hz are most often used. Flexor carpi radialis H-reflexes may be used to monitor median peripheral nerve, brachial plexus, and segmental sensory and motor spinal nerve root and spinal cord function. These reflexes can also be used to monitor the function of a variety of suprasegmental descending spinal cord systems that control the C6/C7 segmental interneurons. The ability to record flexor carpi radialis H-reflexes may be affected by pre-existing abnormalities, such as a generalized polyneuropathy, plexopathy, or radiculopathy. H-reflexes may be absent, latencies may be prolonged, amplitudes may be decreased, and the CMAP configuration may change. Amplitudes may also be decreased with the presence of a myopathy process [1, 2].
Anesthetic Technique
To record reflexes intraoperatively, it is critical that the anesthetic technique does not significantly inhibit the activity of suprasegmental spinal cord function, spinal interneurons, and segmental motor neurons. Also, adequate neuromuscular junction (NMJ) transmission must be present. Reflexes and F-responses are usually monitored during the recording of SSEPs, tcMEPs, EEG, and free-run EMG. The anesthetic technique used must allow for the recording of all these signals with maximum sensitivity (see Chap. 19 for a discussion of general anesthesia monitoring) [2, 55–60].
The technique used varies depending on the surgical procedure, patient’s age, medical history, and the presence of a pre-existing neurologic deficit. Frequently, total intravenous anesthesia (TIVA) with constant infusion of propofol and fentanyl and other opioids are used. Bolus injections of these agents should be avoided to prevent suppression of recordings and if nitrous oxide is used it should not exceed 50 vol.% [61]. Ketamine may serve an adjunctive role to reduce propofol utilization. High dose of ketamine may suppress tcMEP amplitudes [58]. Ketamine is an excitatory agent and increases SSEP, tcMEP, and H-reflex amplitudes [62].
Dexmedetomidine can be used to supplement propofol during TIVA. Suggested therapeutic levels (0.5–0.7 μg/kg/h) suppress MEP amplitudes but not SSEP amplitudes [63, 64]. One study found that 0.6 μg/kg/h did not suppress SSEP, tcMEPs, and visual evoked potentials [65]. Subtherapeutic doses of less than 0.5 μg/kg/h combined with propofol do not suppress tcMEPs [66, 67].
The author studied the effect of dexmedetomidine on intraoperative SSEPs, tcMEPs, EEG, H-reflexes, and F-responses in 17 patients during correction of scoliosis. After completion of distraction and fixation, a loading dose of 1 μg/kg of dexmedetomidine was added to the fentanyl and propofol technique and this was followed by an infusion of 0.5 μg/kg/h to the end of surgery. No muscle relaxants were used. The addition of dexmedetomidine had no effect on SSEPs or EEG but the effect on motor signals was variable. Lower extremity tcMEP amplitudes decreased from 0 to 100 % (mean, 86 ± 16.6 %), F-response amplitudes decreased from 0 to 100 % (mean, 58 ± 12.5 %), and H-reflex amplitudes decreased from 0 to 100 % (mean, 71 ± 13.9 %). Because of the variable and at times pronounced effects on motor signals, it was recommended that dexmedetomidine not be used to monitor tcMEPs, H-reflexes, or F-responses [68].
NMJ-blocking agents should be avoided other than using short-acting agents for intubation. NMJ function needs to be carefully monitored. The most common technique is the train-of-four (TOF) response . Another technique is the T1 % response. T1 % = first unblocked response/control response × 100. NMJ monitoring should be conducted on muscles that may be affected by the surgical procedure.
The effects of propofol on the soleus H-reflex were studied in 33 patients. No NMJ-blocking agents were used. H-reflexes were recorded before administration of anesthetics and after an initial dose of 2 mg/kg over 1 min followed by a continuous infusion at a rate of 167 μg/kg/min for 10 min. Measurements were also made after infusing propofol to a blood level of 6 and 9 μg/mL. The initial dose of 2 mg/kg decreased the H-reflex amplitude and H:M ratio. The following 10-min infusion did not further decrease these values. The 6-μg/mL injection did not change the H-reflex amplitude and H:M ratio. The 9-μg/mL injection decreased the amplitude and H:M ratio. They recommended that the propofol induction dose be 1.0–2.5 mg/kg and this should be followed by an infusion from 100 to 200 μg/kg/min. They concluded that the immobility during propofol anesthesia is not caused by a depression of spinal motor neuron circuit excitability. Propofol does not decrease axon conduction peripherally nor transmission at the neuromuscular junction [69].
Soleus H-reflexes were used to determine the level of motor neuron excitability intraoperatively. In ten normal human volunteers, 1.0–1.5 % enflurane was found to decrease H-reflex amplitudes from 35 to 100 % of baseline values [70].
The effect of isoflurane alone and isoflurane with N20 on the soleus H-reflex was studied under general anesthesia in 25 patients. No NJM-blocking agents were used. Twenty-three of these patients had consistently measurable stable H-reflexes with baseline amplitudes varying from 3.43 to 11.97 mV. The addition of 0.68 % isoflurane decreased the amplitude to 48 % of the baseline. Increasing the isoflurane to 1.37 % decreased the amplitude to 33.8 % of baseline. The combination of 0.81 % isoflurane with 30 % N20 decreased the amplitude to 66.2 % of baseline. The combination of 0.37 % isoflurane with 70 % N20 decreased the amplitude to 30.4 % of the baseline. They reported that increasing isoflurane concentration results in decreased H-reflex amplitude. Increasing the N20 concentration results in decreased H-reflex amplitude. They concluded that the H-reflexes may be recorded with a combination of isoflurane and N20. The H-reflex is maximally stable within the range of anesthetic concentrations used to achieve surgical immobility [55].
The effect of isoflurane and N20 on spinal motor neuron excitability was studied in eight adult patients by monitoring soleus H-reflexes and abductor hallucis F-responses. No NMJ-blocking agents were used. H-reflex amplitude was decreased to 48.4 ± 18.6 % of the baseline with 0.6 minimum alveolar concentration (MAC) isoflurane and to 33.8 ± 19.1 % with 1.2 MAC isoflurane. MAC is defined as the alveolar concentration of an anesthetic agent that prevents movement in 50 % of patients in response to a painful stimulus. F-response amplitude and persistence decreased to 52.2 ± 22.8 % and 44.4 ± 26.0 % of the baseline at 0.6 MAC isoflurane and to 33.8 ± 26.0 % and 21.7 ± 22.8 % at 1.2 MAC. With 1.0 MAC isoflurane, the H-reflex amplitude was decreased by 32.5 ± 19.2 %, 33.3 ± 20.8 %, and 30.4 ± 23.5 % of baseline levels at 30, 50 and 70 % nitrous oxide, respectively [71].
In 12 adult patients, a comparison of the effects of isoflurane on tcMEPs and F-responses were studied. Anesthesia was maintained with 60 % N20, 100 μg/kg/min of propofol and supplementary fentanyl, 0.5–1.0 μg/kg. Recordings were made before and after adding 0.5 % isoflurane. Baseline tcMEP amplitudes (median, 205 μV, 25th–75th percentiles, 120–338 μV), F-response amplitudes (median, 100 μV, 25th–75th percentiles, 64.2–137.5 μV) and F-response persistence (59 ± 29 %) were decreased to 0.0 μV (0–15 μV), 49 μV (12.4–99.6 μV), and 30 ± 31 %, respectively, by 0.5 % isoflurane. The tcMEPs were suppressed more than the F-responses. No NMJ-blocking agents were used [72].
Soleus H-reflexes and abductor hallucis F-responses were monitored to determine the effect of hyperventilation and hypoventilation on motor neuron excitability during isoflurane anesthesia. H-reflex and F-responses were recorded before and after changing the end-tidal CO2 (ETCO2) concentration. Anesthesia was maintained with 0.8 % isoflurane and no muscle relaxants were used. An ETCO2 of 25 mmHg decreased the preanesthetic H-reflex amplitude from 6.8 ± 2.7 mV to 4.0 ± 2.0 mV and to 2.0 ± 2.2 mV at an ETCO2 of 45 mmHg. F-response persistence decreased from the preanesthetic value of 100 % to 77 % ± 24 % at an ETCO2 of 25 mmHg and to 61 ± 19 % at an ETCO2 of 45 mmHg. They concluded that hyperventilation and hypoventilation effects motor neuron excitability and may affect the probability of patient movement during surgery [73].
Volunteers were sedated with a constant level of propofol (2 mg/L) and to a constant level of sevoflurane (0.8 vol.%). Soleus H-reflexes were used to study the depressive effects of these agents individually. The amount of depression was found to be dependent on the size of the H-reflex and is different at different stimulus intensities, indicating a varying effect of propofol and sevoflurane on motor neurons of different size. H-reflex depression is more for both agents at a lower intensity of stimulation than at a higher intensity of stimulation, indicating that smaller motor neurons are depressed more than larger motor neurons. In contrast, excitability of motor neurons by supraspinal effects affects the larger before smaller motor neurons [74]. From a practical standpoint, if H-reflexes are being recorded with a low stimulus intensity and injury occurs that effects the larger motor neurons, this injury could be missed because only the function of the smaller motor neurons would be monitored. The smaller motor neuron population would already be suppressed by the anesthesia, further decreasing the sensitivity for detecting injury. A higher intensity of stimulation would activate small and large motor neurons, allowing the monitoring of a greater percentage of the motor neuron pool.
Clinical Correlation of H-Reflexes and F-Responses
Studies of the normal and abnormal electrophysiology of the spinal cord CPG components in humans and animals have resulted in the understanding of the mechanisms involved in acute complete and partial SCI in humans. How H-reflex and F-response changes correlate with the patient’s postoperative status is derived from the publications discussed as follows.
Soleus H-reflexes and abductor hallucis F-responses were recorded in 32 patients during spinal cord surgery. In six patients, an abrupt fall in H-reflex amplitude beyond 3 SD from the baseline or significant drop in F-response persistence coincided to perturbation or injury to the spinal cord. Transient suppression occurred in four patients with less than a 50 % drop in H-reflex amplitude or abductor hallucis F-response persistence. These patients did not develop a new postoperative neurologic deficit. Suppression exceeded 90 % of the baseline values and persisted through surgery in two patients. Both patients had profound postoperative neurologic deficits. The author concluded that rostral SCI suppresses H-reflexes and F-responses and the degree of suppression reflects the severity of injury. The mechanism responsible for these changes is thought to be hyperpolarization of caudal motor neurons, which occurs within seconds of injury [75].
H-reflexes were recorded in the lower extremities in 31 patients during spinal surgery. A significant change in amplitude was considered significant if it exceeded 3 SD of the mean postanesthetic baseline. In six patients, a significant decrease in H-reflex amplitude occurred. The onset of H-reflex suppression coincided with a potentially injurious event in each case. In one case involving a cervical myelotomy, a large syrinx collapsed during decompression, producing immediate fluctuation in the H-reflex amplitude. The amplitude recovered to the baseline level 9 min later. Postoperatively, no neurologic deficit was noted. Another case involved mechanical reduction of a T-8 spinal fracture. Increased variability in H-reflexes developed with manipulation of the spine before reduction. The first attempt to reduce the fracture produced a transient fall in H-reflex amplitude . The second attempt at reduction produced a pronounced reduction in amplitude to less than 10 % of baseline. H-reflexes remained suppressed until the end of surgery and postoperatively the patient had severe motor and sensory deficits in both legs that were not present preoperatively. A third case involved a cervical myelotomy for decompression of a posttraumatic syrinx.
During surgery, cervical cord hemorrhage occurred and was followed by reduction H-reflex amplitude. The amplitude steadily declined and the H-reflexes disappeared and remained absent. Postoperatively, the patient had profound weakness in both lower extremities. The changes in these patients demonstrated that H-reflex changes occur immediately at the time of spinal injury. H-reflex changes may be reversible and reflect the severity of SCI [4].
H-reflexes may indicate intact spinal cord function with changes in SSEPs. Soleus H-reflexes and tibial SSEPs were monitored in a patient during T7–T12 laminectomy for spinal stenosis. During laminectomy, the left SSEP became absent and the right amplitude was significantly transiently reduced. No H-reflex changes occurred. Postoperatively, no lower extremity motor deficits were present and no new sensory deficits [76].
Spinal cord function was monitored in 278 pediatric spine with gastrocnemius H-reflexes and SSEPs. Combined H-reflex and SSEP monitoring improved the reliability for detecting spinal cord compromise compared with either procedure alone. H-reflexes changed more than SSEPs. The changes reflected changes in spinal cord gray matter function related to acidosis and changes in hematocrit and blood pressure [77].
In a clinical setting, soleus H-reflexes and abductor halluces F-responses were recorded in 14 patients following SCI that resulted in either partial injury without spinal shock or injury with spinal shock. Deep tendon reflexes following tap of the Achilles tendon and patellar tendons were also evaluated. Patients were evaluated within 24 h of injury and on day 10, 20, and 30 after injury. F-responses were absent in patients with spinal shock, reduced in persistence in patients with acute injury without spinal shock, and normal in persistence in patients with chronic injury. F-response changes persisted up to 2 weeks following SCI. H-reflexes were absent or markedly suppressed in patients with spinal shock within 24 h of injury but recovered to normal amplitude within several days of the injury. Deep tendon reflexes were proportionally more depressed in spinal shock than H-reflexes. This demonstrated dissociation between electrically and mechanically induced reflexes during spinal shock . The observation that the stretch reflex is more depressed than the H-reflex is consistent with depressed fusimotor drive with SCI [19].
A 63-year-old woman with a large T8–9 herniated disk that was causing spinal stenosis with cord compression was treated with a bilateral T8–9 laminectomy with left far lateral costovertebral exposure for disk removal. She presented clinically with right T8 dermatomal and bilateral lower extremity pain. Baseline intraoperative tibial SSEPs were bilaterally normal. Baseline lower extremity tcMEPs were present. Baseline lower extremity H-reflexes and F-responses were present bilaterally. During removal of the calcified disk, the lower extremity tcMEPs became absent. The left vastus medialis H-reflex became absent and the left tibialis anterior, gastrocnemius, and abductor hallucis H-reflex amplitudes were decreased 47, 61, and 97 %, respectively. The right tibialis anterior, gastrocnemius, and abductor hallucis H-reflex amplitudes were decreased 75, 88, and 97 %, respectively. The F-responses were present with 35 % of the stimuli on the left and with 30 % of the stimuli on the right. Baseline F-responses were present bilaterally with each stimulus. There were no changes in the tibial SSEPs. Postoperatively, the patient’s lower extremity sensory function was normal. The lower extremity pain was gone but pain was still present in the right T8 distribution. The lower extremities were weak; she could not stand and could not perform coordinated lower extremity functions. Her strength improved and she was able to walk with assistance on discharge 12 days after surgery. The 1.9 to 8.4 % [78, 79] of the motor neuron pool activated by transcranial electrical stimulation was lost. The 24–100 % [20] of the motor neuron pool activated by the H-reflexes was decreased from 47 to 100 %. The 1.0–5.0 % [21] of the motor neuron pool activated by the F-responses was deceased by 65 and 70 %. The pattern of change in these motor systems in this patient indicates that these techniques may activate the same, different, or overlapping populations of the motor neuron pool. Since the tcMEPs became absent and there was preservation of some H-reflex and F-response function, perhaps H-reflex and F-response changes are better predictors of postoperative motor function than are tcMEPs. The H-reflex and F-response changes correlated with the patient’s postoperative motor function while the tcMEPs did not [80].
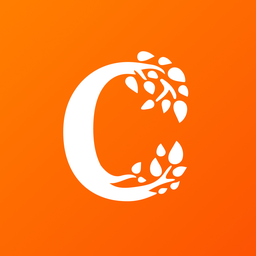
Full access? Get Clinical Tree
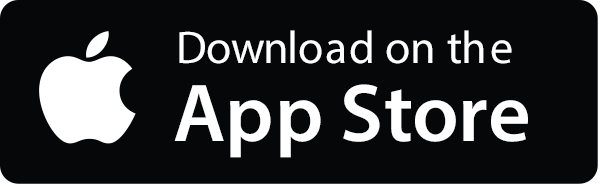
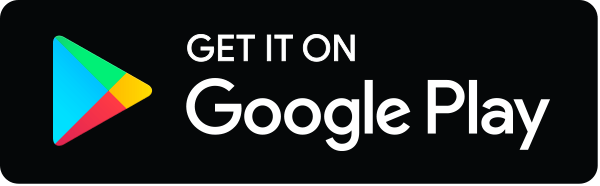