Abstract
The placenta is a critical organ of great importance to obstetric anesthesia. Revered by ancient cultures as “the seat of the external soul” or “the bundle of life,” the placenta is involved in many cultural rituals. Acknowledgment and understanding of the indispensable role of the placenta continues to evolve. The notion of the placenta as a passive conduit for oxygen, nutrients, and waste has been dispelled with the realization that the placenta is a complex and dynamic organ that serves critical functions of metabolism, nutrition, and hormonal maintenance during pregnancy. Maternal-placental conditions can affect the fetus during pregnancy with consequences into adulthood and even beyond into the next generation.
The placenta brings the maternal and fetal circulations into close apposition without substantial interchange of maternal and fetal blood for the physiologic transfer of gases, nutrients, and wastes. This important exchange is accomplished within a complex structure that is almost entirely of fetal origin.
Keywords
Placenta, Development, Physiology, Drug transfer, Epigenetic, Anesthetic implications, Disease state
Chapter Outline
Anatomy, 56
Physiology, 60
Barrier Function, 60
Hormonal Function, 60
Regulation of Placental Blood Flow, 60
Transport Mechanisms, 60
Transfer of Respiratory Gases and Nutrients, 61
Drug Transfer, 63
Pharmacokinetic Principles, 63
Inhalation Anesthetic Agents, 64
Induction Agents, 64
Dexmedetomidine, 66
Benzodiazepines, 66
Opioids, 66
Nonopioid Analgesics, 67
Local Anesthetics, 67
Muscle Relaxants, 67
Anticholinergic Agents, 67
Anticholinesterase Agents, 67
Sugammadex, 67
Antihypertensive Agents, 67
Vasopressor Agents, 68
Anticoagulants, 68
Drug Delivery Systems, 69
Disease States, 69
Infection and Inflammation, 69
Placental Pathology, 70
The placenta is a critical organ of great importance to obstetric anesthesia. Revered by ancient cultures as “the seat of the external soul” or “the bundle of life,” the placenta is involved in many cultural rituals. Acknowledgment and understanding of the indispensable role of the placenta continues to evolve. The notion of the placenta as a passive conduit for oxygen, nutrients, and waste has been dispelled with the realization that the placenta is a complex and dynamic organ that serves critical functions of metabolism, nutrition, and hormonal maintenance during pregnancy. Maternal-placental conditions can affect the fetus during pregnancy with consequences into adulthood and even beyond into the next generation.
The placenta brings the maternal and fetal circulations into close apposition without substantial interchange of maternal and fetal blood for the physiologic transfer of gases, nutrients, and wastes. This important exchange is accomplished within a complex structure that is almost entirely of fetal origin.
Anatomy
Embryology
The blastocyst attaches to endometrial pinopodes (uterodomes), which express markers of endometrial receptivity (e.g., galectin-9), and serine proteases and metalloproteinases (e.g., MMP-2 and MMP-9) initiate the remodeling of the uterine extracellular matrix. The syncytiotrophoblasts (invasive cells located at the margin of the growing conceptus), with the assistance of endometrial stromal cells, invade the decidua, capillaries, and arterioles until the blastocyst is surrounded by circulating maternal blood (trophoblastic lacunae). The vitelline vein system develops in the embryonic yolk sac to enhance nutrient transport from the maternal blood through the trophoblast layer and chorionic plate into the chorionic cavity. The embryo undergoes a dramatic acceleration in growth as its dependence on simple diffusion diminishes.
At 2 weeks of development, the primitive extra-embryonic mesoderm (cytotrophoblast layer) begins to proliferate as cellular columns, carrying a syncytiotrophoblast covering and extending into the maternal blood lacunae; these represent primary villi. Development within the core of these primary villi marks the metamorphosis into secondary villi. Further cellular differentiation of the villi mesoderm into blood cells and vessels marks the transition into tertiary villi. Each villus develops connections within the chorionic plate and into the stalk connecting the developing embryo with the primitive placenta. Penetration of the cytotrophoblast continues through the syncytiotrophoblastic layer until many of the villi reach the decidua and form anchoring villi ( Fig. 4.1 ). Villi undergo extensive branching into the lacunar (or intervillous) spaces, enlarging the surface area available for exchange. Villous maturation reduces the cytotrophoblast thickness, thereby shortening the distances required for diffusion.

The embryo attaches to the chorion via a connecting body stalk. Mesodermal components of this stalk coalesce to form the allantoic (or rudimentary umbilical) vessels. The expansive open region at the ventral surface of the embryo constricts as the body wall grows, surrounding the yolk stalk, allantois, and developing vessels into a primitive umbilicus. The mature umbilical cord forms as the expanding amnion surrounds the connecting stalk and yolk sac.
Placental development is a dynamic process influenced by many factors. Nitric oxide plays an important role in embryo development, implantation, and trophoblast invasion in diverse species. Human endothelial nitric oxide synthase (eNOS) expression in the syncytiotrophoblast and early endothelium occurs in the first trimester and becomes more prominent throughout pregnancy. Angiogenesis depends on vascular endothelial growth factor (VEGF) and its receptors VEGFR-1 (Flt-1) and VEGFR-2 (Flk-1), transforming growth factor-β 1 (TGF-β 1 ), and angiopoietin 1 and 2, which exert their effects partially through nitric oxide. Hypoxia also plays an important role in placental development and angiogenesis by stimulating trophoblast invasion and differentiation via hypoxia-inducible factor-alpha, which activates VEGF and eNOS. Relative hypoxia must be maintained in early placental development because the placental-fetal unit cannot tolerate the oxidative stress of reactive oxygen species during organogenesis. Oxygen levels influence the placental vascular sensitivity to vasodilators and vasoconstrictors. In vitro studies have shown that NOS inhibition and hypoxia independently increase placental perfusion pressure. Both of these effects are prevented by nitric oxide donors, suggesting a common pathway with the effect of hypoxia mediated partly by low NOS activity.
Preeclampsia is related, at least in part, to abnormal placental growth and implantation at early stages of development, resulting in the villous tree having longer capillaries with fewer branches (see Chapter 35 ). Vascular dysfunction occurs mainly from changes in vascular structure and synthesis of nitric oxide rather than from altered responses to nitric oxide and vasoconstrictors.
The expression and control of DNA and genes affects placental development, fetal development, adult phenotype expression, and clinical diseases, even into subsequent generations. Epigenetics explores the prolonged effect of maternal and paternal environmental influences; gene expression becomes altered by DNA methylation, histone modification, and noncoding RNA. At fertilization, global DNA methylation is erased so the genome is hypomethylated at the blastocyst stage (implantation). Methylation of placental DNA increases with gestation, causing down- and up-regulation of genes associated with cell cycles and immune response, respectively. Genomic imprinting causes the silencing of one allele-specific copy of a gene, which leads to extensive intraplacental and cotyledon mosaicism. In vitro fertilization, diet, and maternal diseases can influence epigenetic changes and subsequently alter DNA methylation.
Altered gene methylation has been linked to clinical disease states. Increased long interspersed nuclear element-1 (LINE1) gene methylation, as well as hypomethylation of 34 specific genes, are associated with early-onset preeclampsia. By contrast, late-onset preeclampsia is associated with hypomethylation of only 4 genes. These and other findings indicate that DNA and DNA regulatory changes influence early placental development as well as the occurrence of pregnancy-associated diseases.
Human studies have demonstrated fetal programming of childhood and adult diseases. Adults who were exposed in utero to episodes of malnutrition developed reduced glucose tolerance, atherogenic lipid profiles, and a doubled rate of cardiovascular diseases; these disease states were associated with hypomethylation of regulatory areas for insulin-like growth factor-2 and other genes. In utero exposure to a high-fat diet can lead to an increased incidence of diabetes in offspring, and chronic maternal stress during pregnancy can lead to infant neurodevelopmental disorders.
The placenta grows dramatically from the third month of gestation until term, with a direct correlation between placental growth and fetal growth. By term, the mature placenta is oval and flat, with an average diameter of 18.5 cm, weight of 500 g, and thickness of 23 mm. At term, the human fetal-placental weight constitutes 6% of maternal weight. The growth of the placenta and fetus is influenced by maternal anabolic status, placental growth hormone, insulin-like growth factor-1, leptin, and glucocorticoids. Whether maternal or fetal in origin, increased glucocorticoids signal adverse environmental conditions and result in reduced glucose and amino acid transfer to the fetus. With 12 subtypes of glucocorticoid receptors, significant variations in placental-fetal development can occur, including alterations in placental remodeling, trophoblastic invasion, and angiogenesis inhibition. Competition between the mother and fetus for resource allocation has been termed the kinship theory, in which imprinted genes influence the balance of nutrient allocation in a context-specific manner.
Comparative Anatomy
Placentas of different species vary in their method of uterine attachment (adhesion, interdigitation, and fusion) and the number of tissue layers between the maternal and fetal circulations. The placental categorization system, called the Grossner classification, uses the number of tissue layers in the placental barrier to help differentiate species ( Fig. 4.2 ).

The ability of the placenta to transfer various substances differs among species. The markedly thicker epitheliochorial placenta found in sheep, a species commonly used for placental transfer studies, has three maternal layers (epithelium, connective tissue, and endothelium) that separate maternal from fetal blood. By contrast, the human hemochorial placenta lacks these maternal layers, which allows maternal blood to bathe fetal tissues directly (see Fig. 4.2 ). As a result, species differ in the transfer of substances through the placenta.
Vascular Architecture
Maternal
Under the initial hormonal influences of the corpus luteum, the uterine spiral arteries elongate and coil. Erosion of the decidua induces lateral looping of the already convoluted spiral arteries. In late pregnancy, approximately 200 spiral arteries can supply approximately 600 mL/min of blood flow directly to the fetus through the placenta. The vasodilation required to accommodate the increased flow is accomplished by the replacement of the elastic and muscular components of these arteries by cytotrophoblastic cells, and later by fibroid cells; vessel diameter increases up to 10-fold, while blood velocity and blood pressure decrease. Inadequate uterine spiral artery development contributes to relative ischemia and the development of preeclampsia and fetal growth restriction, which was previously called intrauterine growth restriction (IUGR). Computer modeling of spiral artery flow indicates that high blood velocity in nondilated spiral arteries causes damage to the endothelium and placenta trophoblast.
The intervillous space is a large cavernous expanse that develops from the fusion of the trophoblastic lacunae and the erosion of the decidua by the expanding blastocyst. Folds in the basal plate form septa that divide the space into 13 to 30 anatomic compartments or lobules for efficient transfer of material between maternal and fetal blood flow. Each lobule contains numerous villous trees that are also known as cotyledons or placentomes. The intervillous space of the mature placenta can accommodate 350 mL of maternal blood.
Maternal arterial blood leaves the funnel-shaped spiral arteries and enters the intervillous space. The blood moves into the nearly hollow, low-resistance area, where villi are very loosely packed (the intercotyledonary space), before entering a region of densely packed intermittent and terminal villi where placental exchange predominates ( Fig. 4.3 ). Maternal venous blood collects between neighboring villous trees in an area called the perilobular zone. Collecting veins penetrate the maternal plate at the periphery of the villous trees to drain perilobular blood from the intervillous space.

Fetal
Two coiled arteries in the umbilical cord bring fetal blood toward the placenta. These arteries divide into chorionic arteries that supply the 50 villous trees located in the placental lobules. At the base of each villous tree, the chorionic arteries are considered the main villous stem or truncal arteries (first-order vessels), which in turn branch into four to eight ramal or cotyledonary arteries (second-order vessels); as they pass toward the maternal plate, they further subdivide into ramulus chorii (third-order vessels) and, finally, terminal arterioles. The terminal arterioles lead through a neck region into a bulbous enlargement, where they form two to four narrow capillary loops. Here the large endothelial surface area and the near-absence of connective tissue allow optimal maternal-fetal exchange ( Fig. 4.4 ).

The venous end of the capillaries loops, narrows, and returns through the neck region to the collecting venules, which coalesce to form the larger veins in the stem of the villous trees, and as they perforate the chorionic plate, become chorionic veins. The venous tributaries combine to empty into the one umbilical vein that delivers blood back to the fetus.
Physiology
Barrier Function
The placenta is an imperfect barrier that allows bidirectional transfer of many substances; the rate and amount of transfer depend on the permeability and the presence of various mechanisms to restrict movement. Many substances undergo specific or nonspecific binding within the placental tissues, thereby minimizing fetal exposure and accumulation; other substances are altered by the vast array of inducible and constitutive cytochrome P450 isoenzymes and transporters. Placental membrane thickness, which may influence the rate of diffusion, diminishes as gestation progresses ; however, the transfer rate of certain simple substances (e.g., glucose, water) differs very little among species, despite significant variations in placental thickness.
Maternal and fetal cellular components can cross the placental barrier by disruption of the trophoblastic layer or by active adhesion and transmigration (similar to blood-brain barrier migration). Fetal cells may be pluripotent, and their DNA can be found in maternal organs for decades. Murine fetal progenitor cells have been found to migrate and assist with maternal wound healing. These microchimeric fetal cells may contribute to maternal immunomodulation, development or worsening of autoimmune diseases (e.g., thyroiditis, lupus, and asthma), and healing of wounds, including neuronal tissue. Placental exosomes, nanovesicles 30 to 100 nm in size found in maternal circulation that contain proteins and transcription-related materials, exert a maternal immunosuppressive effect. Placental microparticles, which are vesicular products of syncytiotrophoblast greater than 100 nm, also contain RNA and DNA fragments and affect fetal and maternal apoptosis, angiogenesis, and inflammation. Syncytial nuclei from the placental villous tree also enter the maternal circulation, reside in the lung, participate in maternal-fetal signaling, and assist in the delivery of retroviral proteins for immunomodulation. An excess of microparticles has been observed in early-onset preeclampsia.
Hormonal Function
A sophisticated transfer of precursor and intermediate compounds in the maternal-fetal-placental unit allows placental enzymes to convert steroid precursors into estrogen and progesterone. This steroidogenic function of the placenta begins very early in pregnancy; by 35 to 47 days after ovulation, the placental production of estrogen and progesterone exceeds that of the corpus luteum (i.e., the ovarian-placental shift).
The placenta also produces and stores a wide array of enzymes, binding proteins, and polypeptide hormones. For example, the placenta produces human chorionic gonadotropin, human placental lactogen (a growth hormone also known as human chorionic somatomammotropin), and factors that control hypothalamic function. Production of proteins and steroid hormones allows the placenta to influence and control the fetal environment.
Regulation of Placental Blood Flow
Maternal Blood Flow
Maternal blood enters the intervillous cotyledon space at a pressure of 70 to 80 mm Hg in an area that has relatively few villi. The pressure and velocity of blood flow rapidly diminishes to 10 mm Hg as blood passes into an area of higher resistance created by the densely packed villi of the placentome.
Fetal Blood Flow
In contrast to the maternoplacental circulation, which relies on vasodilation to increase flow, the increase in fetoplacental blood flow is primarily caused by vascular growth. Fetal perfusion of the placenta is not classically autoregulated; the placental vasculature is not innervated by the sympathetic nervous system. However, the fetus can modulate fetoplacental perfusion via: (1) endocrine effects of adrenomedullin, (2) net efflux/influx of water regulated by fetal blood pressure, and (3) local autoregulatory effects mediated by the paracrine vasodilators nitric oxide and acetylcholine. Adrenomedullin release by the fetal adrenal glands assists in maintenance of tone in placental vessels. Fetal blood pressure changes cause net influx/efflux of water across the placenta that affects fetal intravascular volume and perfusion. Maternal hyperglycemia and hypoxemia can alter regional fetal blood flow, probably through vascular mediators. Endothelium-derived relaxing factors, especially prostacyclin and nitric oxide, are important in the control of fetoplacental circulation. Hypoxia-induced fetoplacental vasoconstriction is mediated by a reduction in the basal release of nitric oxide. This vasoconstrictor activity is functionally similar to that found in the lung (i.e., hypoxic pulmonary vasoconstriction) and allows optimal fetal oxygenation through redistribution of fetal blood flow to better-perfused lobules. The placental vasculature constricts in response to graded hypoxia, and may be more dependent upon angiotensin II than catecholamines.
Transport Mechanisms
Substances are transferred across the placenta by one of several mechanisms.
Passive Transport
The passive transfer of molecules across a membrane depends on (1) concentration and electrochemical differences across the membrane, (2) molecular weight, (3) lipid solubility, (4) degree of ionization, and (5) membrane surface area and thickness. Passive transfer is driven principally by a concentration gradient and occurs through the lipid membrane (e.g., lipophilic molecules and water) or within protein channels that traverse the lipid bilayer (e.g., charged substances such as ions). Drugs with a molecular weight less than 600 Da cross the placenta by passive diffusion.
Facilitated Transport
Carrier-mediated adenosine triphosphate (ATP)–independent transport of relatively lipid-insoluble molecules down their concentration gradient is called facilitated diffusion. Facilitated diffusion differs from simple diffusion in several ways. Specifically, this mode of transfer exhibits (1) saturation kinetics, (2) competitive and noncompetitive inhibition, (3) stereospecificity, and (4) temperature influences (e.g., a higher temperature results in greater transfer). With simple diffusion, the net rate of diffusion is proportional to the difference in concentration between the two sides of the membrane. The rate of transfer is determined by the number of membranous carrier protein complexes and the extent of interaction between the carrier and the substance undergoing transport. This rate limitation is valid for facilitated diffusion only when transmembrane concentration differences are small. At higher concentration gradients, a maximum rate of transfer (V max ) is reached; thereafter, further increases in the concentration gradient do not affect the rate of transfer. An example of facilitated diffusion is the transplacental transfer of glucose.
A special type of facilitated diffusion involves the “uphill” transport of a molecule linked to another substance traveling down its own concentration gradient. As such, the transfer is not directly driven by cellular energy expenditure. In most cases, sodium is the molecule that facilitates transport. For the membrane-bound carrier to transfer these molecules, both molecules must be bound to the carrier. This hybrid system is called secondary active transport or co-transport. The transplacental transport of amino acids appears to occur principally through secondary active transport. Transporters may be affected by disease states (e.g., preeclampsia) or signaling molecules (e.g., elevated steroid levels).
Active Transport
Active transport involves the movement of any substance across a cell membrane requiring energy from ATP hydrolysis. In general, active transport occurs against a concentration, electrical, or pressure gradient. Active transport also requires a protein membrane carrier that exhibits saturation kinetics and competitive inhibition. The best-known example of primary active transport is the translocation of sodium and potassium through the sodium-potassium adenosine triphosphatase (Na + /K + ATPase) pump. Unlike most cell types, the placenta can self-generate creatinine, which assists in the regeneration of ATP and in meeting the high metabolic energy demands of the placenta.
Active transport proteins include P-glycoprotein, breast cancer resistance protein, multidrug resistance protein, and the sodium/multivitamin transporter, as well as the many proteins involved in monoamine transport and xenobiotics. These transport proteins play an important role in protecting the fetus from foreign and potentially teratogenic compounds. Drugs may compete with endogenous compounds of similar shape and charge for active transport. P-glycoprotein transports many lipophilic drugs and antibiotics and removes cytotoxic compounds from the fetus; it exists on the maternal side of the trophoblastic cell membrane of the placenta and prevents compounds such as methadone and saquinavir (a protease inhibitor) from leaving the maternal blood, thus limiting fetal exposure. Inhibition of these transporter proteins (e.g., inhibition of P-glycoprotein by verapamil) can significantly increase the fetal transfer of certain drugs, including midazolam, which is a substrate for P-glycoprotein. DNA transcription of transporters may be induced by drugs or disease states. Expression of transporters may change with gestational age.
Pinocytosis
Pinocytosis is an energy-requiring process in which the cell membrane invaginates around large macromolecules that exhibit negligible diffusion properties. Although the contents of pinocytotic vesicles are subject to intracellular digestion, studies have demonstrated that the vesicles can move across the cytoplasm and fuse with the membrane at the opposite pole. This appears to be the mechanism by which immunoglobulin G is transferred from the maternal to the fetal circulation.
The placenta releases extracellular vesicles of various sizes and functions. Macrovesicles from the syncytiotrophoblast (20 to 500 µm) carry fetal protein, RNA, and other substances; microvesicles from membranes of stressed or apoptotic cells (0.1 to 1 µm) and exosomes (20 to 100 nm) contribute to feto-maternal communication.
Other Factors That Influence Placental Transport
Other factors that affect maternal-fetal exchange include (1) maternal and fetal blood flow, (2) placental binding, (3) placental metabolism, (4) diffusion capacity, (5) maternal and fetal plasma protein binding, and (6) gestational age (the placenta is more permeable in early pregnancy). Lipid solubility, pH gradients between the maternal and fetal environments for some basic drugs (“ion trapping”), and alterations in maternal or fetal plasma protein concentrations found in normal pregnancy and other disease states (e.g., preeclampsia) may also alter net placental transport.
Transfer of Respiratory Gases and Nutrients
Oxygen
The placenta must provide approximately 8 mL O 2 /min/kg fetal body weight for fetal growth and development, while adults only require 3 to 4 mL O 2 /min/kg at rest. As the “lung” for the fetus, the placenta has only one-fifth the oxygen transfer efficiency of the adult lung. The human lung, with a surface area of 50 to 60 m 2 and a thickness of only 0.5 µm, has a very large oxygen diffusion capacity; in comparison, the placenta has a lower diffusion capacity because of its smaller surface area of 16 m 2 and a thicker membrane of 3.5 µm. Furthermore, 16% of uterine blood flow and 6% of umbilical blood flow are shunted through the placenta.
Oxygen transfer across the placenta depends on the membrane surface area, membrane thickness, oxygen partial pressure gradient between maternal blood and fetal blood, oxygen affinity of maternal and fetal hemoglobin, and relative maternal and fetal blood flows. As physically dissolved oxygen diffuses across the villous membranes, bound oxygen is released by maternal hemoglobin in the intervillous space and diffuses across the placenta. Several factors affect the fetal blood P o 2 once it reaches equilibration in the villi end-capillaries. First, the concurrent and countercurrent arrangements of maternal and fetal blood flow play a key role for placental oxygen transfer in various species. The almost complete equilibration of maternal and fetal P o 2 values suggests that a concurrent (or parallel) relationship between maternal blood and fetal blood exists within the human placenta ( Fig. 4.5 ), although others have described a multivillous, cross-current flow pattern. The functional anatomy of the placenta in many mammals involves more layers than the human placenta; thus, results of animal models can only provide evidence for trends, not values, in humans.
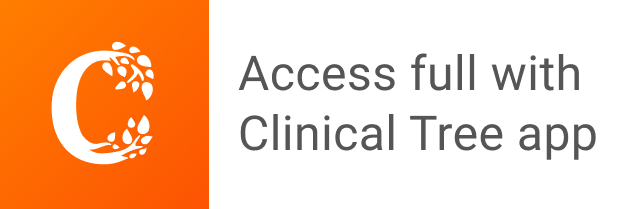