The Electrophysiology of Arrhythmias
Peter J. Kudenchuk
Patient management suffers when health care providers base clinical decisions solely on ECG findings and neglect to evaluate the patient’s clinical condition or correlate symptoms and findings with rhythm disturbances. Similarly, rhythm management suffers when arrhythmias are treated without some understanding of their electrophysiologic mechanisms.
Review of basic electrophysiology to clarify clinical arrhythmias
Mechanisms of arrhythmia generation and propagation
Anatomy and physiology of the conduction system
Relationship of electrophysiology to the surface electrocardiogram (ECG)
Introduction
This chapter presents the basic mechanisms and clinical significance of commonly encountered cardiac rhythm disturbances. To support this understanding, it offers an overview of the cardiac cellular environment and the mechanisms governing the heart’s “electrical cells” and “muscle cells.”
Cell Types
Two groups of cells in the myocardium, muscle (or contractile) and electrical cells, determine the heart’s function as a pump. Muscle cells produce the cardiac contractions by means of elaborate protein-contractile structures within them. They are activated by electrical cells, composed of pacemaker cells and electrical conducting cells, from which electrical activity originates and is then transmitted via specialized conducting pathways in the heart. Muscle (contractile) and electrical cells are intricately interwoven in a manner that facilitates rapid and efficient propagation of electrical impulses from their site of origin in pacemaker cells (such as the sinus node) and their spread via electrical conducting cells (such as the His–Purkinje network) to their final muscle cell destinations throughout the heart. Muscle cells are also electrically active and can conduct electrical impulses from cell to cell, but not as rapidly or efficiently as electrical conducting cells. Proper interactions between the electrical conducting cells and the muscle cells produce repetitive, coordinated contraction/relaxation cycles. These cycles produce an effective cardiac output over a wide range of physiologic conditions and stress.
Charged ions, predominantly calcium, potassium, and sodium, cross myocardial (muscle and electrical) cell membranes back and forth. These ion movements mediate electrical excitation and muscle contraction. Many conditions can alter ionic movement across cell membranes or charged cell components and thereby interfere with coordinated electrical and mechanical function. This interference can produce symptomatic arrhythmias or even sudden cardiac death.
Basic Cardiac Electrophysiology: Cellular Level
Ion Gradients
For a cell to do work or conduct an impulse, the cell’s membrane must be electrically charged. This charge arises from concentrations of potassium, sodium, and calcium inside the cell that vary from those outside the cell. Normally the concentration gradient of ions produces a negative electrical charge across the membrane. When the cell is activated, or depolarized, this negative charge moves rapidly towards a positive membrane charge. This change in membrane charge initiates depolarization (or activation). A similar process results in cell-to-cell spread of this activation, referred to as conduction, which is ultimately electromechanically linked to mechanical contraction.
Depolarization: The Process
With depolarization, there is a momentary change in the physical properties of the cell membrane. Positively charged ions begin to enter the cell through one of two channels, causing the inside to become less negative and ultimately positive:
The fast channel permits rapid entry of sodium ions and is responsible for depolarizing myocardial muscle (contractile) cells.
The slow channel permits entry of calcium ions and possibly sodium ions. Slow channel depolarization produces the spontaneous pacemaker activity of the sinus node, the atrioventricular (AV) junction, and other pacemaker cells of the heart.
Action Potential of a Myocardial Muscle Cell
In a typical ventricular myocardial muscle (contractile) cell, the resting membrane potential is electrically negative compared with the outside of the cell membrane. Sodium exists in high concentration outside the cell and in low concentration inside the cell. Figure 20-1A depicts the change in movement of ions across a myocardial muscle cell membrane as it propagates an action potential. The concentration gradient of the sodium ions and the electrical charge across the membrane provide the driving force for the sodium during the action potential. The cell membrane expends considerable energy to pump sodium ions out of the cell to maintain the concentration gradient.
Phases of the Action Potential
Preparing for Phase 0:
Between contractions, the cell membrane is relatively impermeable to sodium. Potassium, high in concentration inside the cell and low in concentration outside the cell, is able to cross the cell membrane. During phase 3 and to some extent during phase 2 of the action potential, potassium moves across the cell membrane from inside to outside. This outward flow of positive ions causes the interior of the cell to become electrically negative and the exterior of the cell to become positive. The resting membrane potential, therefore, depends primarily on the potassium gradient across the cell membrane. This is also why the transmembrane potential just prior to phase 0 is depicted in Figure 20-1A in the negative millivolt range.
Phase 0: Depolarization
When depolarization starts, the cell membrane’s fast channel opens briefly, for about 1 millisecond. This permits very rapid sodium entry into the cell, as shown by the long Na+ arrow at the cell membrane in Figure 20-1A. The inward flow of positively charged sodium ions across the cell membrane makes the inside of the cell electrically positive relative to the outside of the cell membrane. The transmembrane potential moves rapidly past 0 mV into the positive range. Phase 0 in the atrial muscle mass generates the P wave and in ventricular muscle the QRS complex. This depolarization (phase 0) propagates through the atrial muscle mass, generating the atrial P wave and, in the ventricular muscle mass, the ventricular QRS complex. (Note the parallel ECG tracing at the top of Fig. 20-1A.)
Phase 1: Start of Repolarization
As the fast channel closes, sodium entry slows down (note the smaller Na+ arrows in Fig. 20-1A). Potassium begins its exit from cells, making the electrical charge inside the cell less positive and starting the repolarization process (phase 1).
Phase 2: Isoelectric or Plateau Phase
During phase 2, the action potential is approximately isoelectric (horizontal line in Fig. 20-1A), but the cell remains depolarized. Significant amounts of sodium are no longer entering the cell through the fast channel, whereas calcium (depicted by the Ca2+ arrows in Fig. 20-1A) begins to enter the cell through the slow channel. Phase 2 of the ventricular muscle action potential is reflected by the ST segment of the ECG. When calcium enters the cell, it activates an interaction between actin and myosin filaments in the muscle cells’ sarcomeres. This interaction results in a contraction. The sarcomere functions as the basic contractile unit of the myocardial fiber.
Phase 3: Rapid Repolarization
Phase 3 represents rapid repolarization, during which the inside of the cell returns to negative (note the transmembrane potential drop in Fig. 20-1A). This return to negative is caused by an increased movement of potassium ions (see long K+ arrow in the figure) from inside to outside the cell. Phase 3 in the ventricular muscle action potential is reflected by the T wave. Repolarization is completed at the end of phase 3. The interior of the cell is again approximately -90 mV.
Phase 4: Sodium/Potassium Pump Activation
Now, immediately after the action potential, the distribution of ions across the cell membrane is different from what it was immediately before depolarization started. Because of sodium entry into the cell and potassium loss from the cell during depolarization, a higher concentration of intracellular sodium and a lower concentration of intracellular potassium now exists. Repeated depolarizations without a redistribution of sodium and potassium ions would soon lead to serious impairment of cell function.
Therefore a special pumping mechanism in the cell membrane is activated during phase 4. (Fig. 20-1A represents this sodium/potassium pump with the curved arrows connecting Na+ and K+ at the cell membrane.) This pumping mechanism, which transports sodium ions from inside to outside the cell and brings potassium ions from outside to inside the cell, depends on adenosine triphosphate (see ATP in Fig. 20-1A) as its energy source. Of note, during phase 4 of the muscle cell as described here, the membrane potential remains at a constant level. This differs from phase 4 of specialized pacemaker cells, described in the following section, during which there is a gradual lessening of membrane potential.
The Action Potential of a Pacemaker Cell and Its Phases
Phase 4: Automaticity: Spontaneous Diastolic Depolarization
Whereas a description of the electrical activity of a muscle (contractile) myocardial cell begins with phase 0, a description of the activity of the electrical pacemaker cell best begins with phase 4. The action potential of a pacemaker cell differs significantly from that of a working myocardial cell. Pacemaker cells possess the property of automaticity, meaning that the cells are able to depolarize spontaneously. The most important feature of the pacemaker cell’s action potential is that the membrane potential during phase 4 does not remain at a constant level, as it does in working myocardial cells (see previous section). During phase 4 in pacemaker cells, as depicted in Figure 20-1B, there is a gradual lessening of the resting membrane potential. This occurs because small amounts of calcium and sodium enter the cell during phase 4, while the outward flow of potassium decreases. The resting membrane potential, therefore, becomes less negative—a process called spontaneous diastolic depolarization.
The slope of phase 4 is important in the rate of impulse formation and ultimately heart rate. The steeper the slope, the faster the rate of the pacemaker cell; the more gradual the slope, the slower the rate. Activation of the sympathetic nervous system (or administration of a catecholamine) makes the slope steeper and thereby enhances automaticity. Stimulation of the parasympathetic nervous system (i.e., vagal stimulation) produces the opposite effect. Drugs such as beta-blockers also decrease the rate of spontaneous depolarization, thus diminishing automaticity.
Phase 0: Threshold Potential
Phase 0 begins when the resting membrane potential reaches a critical voltage (the threshold potential) (see Fig. 20-1B). The rate of rise of phase 0 is slower (less steep) than that of a normal myocardial working cell. The rate of action potential rise (slope) in cells of the sinus node and AV junction depends on the rate of calcium ion entry through the slow channel.
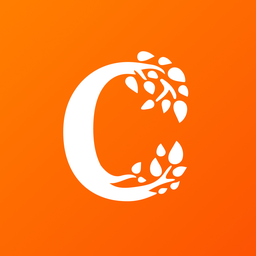
Full access? Get Clinical Tree
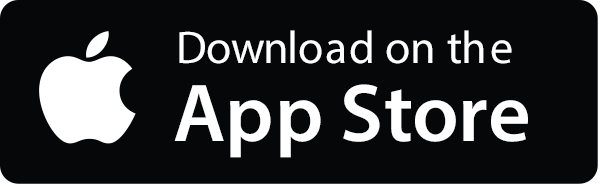
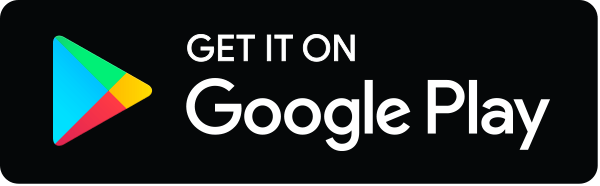
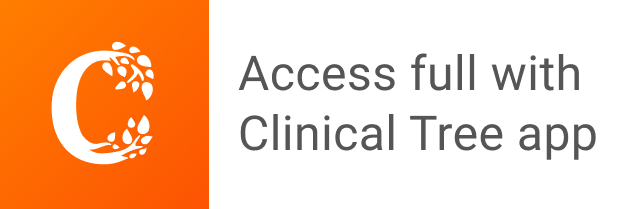