Epidemiology
According to the Central Brain Tumor Registry of the United States (CBTRUS) for the years 2007–2011, the incidence of primary brain and central nervous system (CNS) tumors is 21.42/100,000 per year. For children and adolescents 0–19 years of age, the incidence is 5.42/100,000. The incidence is 27.85/100,000 for adults (> 20 years). For the year 2015, the estimate is 68,470 primary brain and CNS new cases. The overall mortality rate is 4.26/100,000. Approximately 34% of the tumors are malignant. The most common tumor is meningioma (36%) followed by glioblastoma (15%). The broad category glioma represents 28% of all tumors. The 5-years survival rate for malignant brain and CNS tumors is 34%, but only 5% for glioblastoma. The majority of tumors (> 80%) are supratentorial. For all primary brain tumors, the median age of diagnosis is 59 years. From 1985 to 1999, the incidence of primary brain tumors rose modestly (1.1% per year). The exact incidence of brain metastases is unknown but certainly underestimated. In about 25% of patients who die from cancer, central nervous system metastases are detected at autopsy. For the five most common sources of brain metastases (breast, colorectal, kidney, lung, and melanoma), 6% of the patients suffer this complication within 1 year of diagnosis of the primary cancer. Thus, these five cancers probably cause approximately 37,000 cases of brain metastases per year in the United States. Conversely, about 10% of patients with lung cancer present to the physician with symptoms from a brain metastasis.
General Considerations
For patients the problems associated with supratentorial tumors result from local and generalized pressure, whereas for surgeons the difficulties arise during surgical exposure because the brain is particularly susceptible to damage from retraction and mobilization. Anesthesia for supratentorial tumors thus requires an understanding of the pathophysiology of localized or generalized rising intracranial pressure (ICP); the regulation and maintenance of intracerebral perfusion; how to avoid secondary systemic insults to the brain ( Box 11.1 ); the effects of anesthesia on ICP, perfusion, and metabolism; and the therapeutic options available for decreasing ICP, brain bulk, and tension perioperatively. Specific problems include massive intraoperative hemorrhage, seizures, and air embolism in the head-elevated or sitting position or if venous sinuses are traversed. Further questions are how to monitor the brain’s function and environment, and whether to aim for rapid anesthesia emergence or for prolonged postoperative sedation and ventilation. Finally, the concurrence of various intracranial and extracranial pathologic conditions should not be forgotten, such as the presence of cardiovascular or pulmonary disease or—in the case of metastases—the existence of paraneoplastic phenomena and the effects of chemotherapy or radiotherapy. This concept can be summarized as follows:
The anesthetic goal: | To preserve brain from secondary insult |
The anesthetic risk factors: | Hypoxemia, hypercapnia, anemia, hypotension |
The anesthetic actions: | Conserve cerebral autoregulation and CO 2 responsiveness Maximize brain elastance to decrease retractor pressure |
Intracranial
Increased intracranial pressure
Midline shift: tearing of the cerebral vessels
Herniation: falx, transtentorial, trans-foramen magnum, transcraniotomy
Epilepsy
Vasospasm
Systemic
Hypercapnia
Hypoxemia
Hypotension or hypertension
Hypo-osmolality or hyperosmolality
Hypoglycemia
Hyperglycemia
Low cardiac output
Hyperthermia
Pathophysiology of Rising Intracranial Pressure
The main normal intracranial components of the brain (tissue, intravascular blood, cerebrospinal fluid [CSF]) are contained in an unyielding skull. Hence any increase in their volume—or the addition of an abnormal mass—must be compensated by a concurrent reduction in volume of one or more of these components, mainly CSF or blood (the brain is largely incompressible) ( Fig. 11.1 ). The ability of these homeostatic mechanisms to compensate depends not only on the volume of the mass but also on the speed at which it arises: for rapidly expanding masses, the ICP volume curve shifts markedly to the left. Early but limited homeostasis is provided by extracranial shifts of intracranial blood, followed by larger-capacity displacement of CSF—which is ineffective if CSF flow is obstructed. Once these compensatory mechanisms are exhausted, ICP rises rapidly, which is followed by impairment of cerebral circulation and, ultimately, by brain herniation; generally subfalcine (“midline shift”) or transtentorial, the end stage of compensation. This concept can be summarized as follows:
The cornerstone of neuroanesthesia: | Intracranial pressure–volume relationship |
The main goal of neuroanesthesia: | Avoiding intracranial compartment volume increase, especially for cerebral blood volume (anesthetics, mean arterial pressure autoregulation, CO 2 ) |
Anesthetic risk factor: | Administration of hypotonic fluidsMedications that affect cerebral autoregulation |

Volume Effects of Intracranial Tumors
The intracranial volume effects of tumors are due not only to the mass of the tumor itself but also to the surrounding vasogenic brain edema. Such edema, commonly seen on preoperative computed tomography (CT) or magnetic resonance imaging, apparently results from secretory factors that increase vascular permeability in the nearby brain. Peritumoral edema is particularly marked around fast-growing tumors, generally responds well to corticosteroid therapy, and can persist or even rebound after surgery for excision of the tumor. Thus the areas surrounding large tumors suffer from ischemia resulting from compression (cerebral blood flow [CBF]) in peritumoral tissue may be decreased by up to one-third compared with normal tissue). Treatment with steroids such as dexamethasone usually results in dramatic decreases in surrounding brain edema. The emergency and preoperative treatment of peritumoral vasogenic edema is the only good indication for steroid therapy in this context.
The Blood–Brain Barrier and Edema
The blood–brain barrier is also affected by intracranial pathologic conditions. Normally the blood–brain barrier is impermeable to large or polar molecules and variably permeable to ions and small hydrophilic nonelectrolytes. Thus any disruption of the blood–brain barrier permits water, electrolytes, and large hydrophilic molecules to enter perivascular brain tissues, leading to vasogenic brain edema. In this case, leakage—and the resulting brain edema—is directly proportional to the cerebral perfusion pressure (CPP). Vasogenic edema should be differentiated from osmotic edema (caused by a drop in serum osmolality) and cytotoxic edema (secondary to ischemia). Blood osmolality is a critical determinant of cerebral edema because a 19-mmHg pressure gradient across the blood–brain barrier is generated for every milliosmole. In contrast, oncotic pressure plays a minor role. Neuroimaging shows disruption of the blood–brain barrier in many tumors. New strategies are being investigated to improve drug delivery to brain tumors. In the future, it is possible that new treatments to augment blood–brain barrier permeability (osmotic blood–brain barrier disruption, intra-arterial chemotherapy) will interfere with perioperative management.
Intracerebral Perfusion and Cerebral Blood Flow
CBF is regulated at the level of the cerebral arteriole. It depends on the pressure gradient across the vessel wall (which in turn is the result of CPP) and PaCO 2 value (which depends on ventilation) ( Fig. 11.2 ). CBF autoregulation, dominant to ICP homeostasis, keeps CBF constant in the face of changes in CPP or mean arterial pressure (MAP). It does this through alterations in cerebral vasomotor tone (i.e., cerebrovascular resistance [CVR]). Autoregulation is normally functional for CPP values of 50 to 150 mmHg and is impaired by many intracranial (e.g., blood in CSF, trauma, tumors) and extracranial (e.g., chronic systemic hypertension) pathologic conditions. It is also affected by drugs used in anesthesia.

If CPP is inadequate, tissue perfusion will decrease when the lower limit of autoregulation is less than 50 mmHg (if autoregulation is intact). Ischemia results at levels of CBF below 20 mL/100 g/min unless CPP is restored (by increasing MAP or decreasing ICP) or cerebral metabolic demand is reduced (through deepened anesthesia or hypothermia). Increased ICP resulting in reduced CPP is met by cerebral arteriolar relaxation; in parallel, MAP is increased via the systemic autonomic response. As a result, a vicious cycle can be established, particularly in the presence of impaired intracranial homeostasis, as cerebral vessel relaxation increases cerebral blood volume (CBV), thus further raising ICP. In addition, an acute reduction in CPP or MAP tends to acutely increase ICP (the so-called vasodilatory cascade ). Reductions in PaCO 2 induce vasoconstriction, reducing CBF, CBV, and thus ICP. Conversely, hypercapnia increases ICP and should be prevented in the perioperative period. This makes hyperventilation a useful tool for the acute control of intracerebral hyperemia and elevated ICP ( Fig. 11.3 ), as summarized here:
The anesthetic goal: | Hemodynamic stability |
The reason: | Autoregulation takes 30 to 120 seconds to be established; thus sharp MAP fluctuations entrain undesirable CBF, CBV, and ICP changes |
The formulas: | CBF = CPP/CVRCPP = MAP − ICPNormally, ICP < CVP |

Anesthesia and Intracerebral Pressure, Perfusion, and Metabolism
Anesthesia exerts major effects on the intracranial environment through a variety of drug and nondrug effects. These effects are sensitive to the state of the intracranial and extracranial environment (e.g., cerebral compliance, presence or absence of intracranial pathologic condition, general volemic state).
Intravenous Anesthetics
Intravenous anesthetics include barbiturates, propofol, etomidate and ketamine. Apart from anesthesia induction, propofol is being increasingly used for maintenance as a continuous intravenous infusion (often computer controlled). All the intravenous drugs mentioned are cerebral vasoconstrictors that act by depression of cerebral metabolic rate (CMR), except ketamine. Ketamine increases whole brain CBF without changing CMR in healthy volunteers. At subanesthetic doses, ketamine increases regional glucose metabolic rate and CBF. The other agents decrease CBF, CBV, and ICP while leaving autoregulation and vessel reactivity to PaCO 2 intact (see Fig. 11.3 ). , CMR reduction reflects brain activity and is mediated through the electrical but not the basal metabolic activity of the neurons. Hence there is a ceiling effect for CMR reduction at electroencephalogram (EEG) burst suppression ( Fig. 11.4 ). In contrast to volatile anesthetics, propofol has been shown capable of suppressing the cerebrostimulatory effects of nitrous oxide. Etomidate directly inhibits adrenal cortisol secretion for 24 to 48 hours even after a single injection, and its use is often associated with myoclonic (not epileptic) movements.

Volatile Anesthetics
All volatile anesthetics are cerebral vasodilators, but isoflurane, sevoflurane, and desflurane also reduce CMR. A flat EEG is obtained with these three agents at around 2 minimum alveolar concentrations (2 MAC), a concentration at which maximum metabolic depression is achieved. The response of cerebral metabolism to rising concentrations of volatile anesthetics is not linear. The decrease in CMR is steep from 0 to 0.5 MAC and then more gradual up to 2 MAC. The effect of volatile anesthetics on CBF is the result of their vasodilatory properties and flow-metabolism coupling. At low concentrations (< 1 MAC), CBF is lower than in the awake person. But CBV is unchanged with isoflurane and decreased with propofol at comparable concentrations. Among the volatile anesthetics, sevoflurane is the least vasodilating and desflurane the most. The effects of xenon are more complex. This agent decreases CBF in gray matter, particularly in specific brain areas such as the thalamus, the cerebellum, the cingulated gyrus, and the hippocampus, and increases CBF in white matter. It does not impair flow-metabolism coupling.
For the normal brain and volatile concentrations below 1 MAC, PaCO 2 reactivity remains intact, permitting control of vasodilation by hypocapnia. (However, the presence of a pathologic brain condition or use of a high-MAC volatile anesthetic may impair or even abolish PaCO 2 reactivity and autoregulation.)
Nitrous Oxide
Nitrous oxide is cerebrostimulatory, increasing CBF, CMR, and sometimes ICP. Its effect is not uniform throughout the brain but is limited to selected brain regions (basal ganglia, thalamus, insula), changing the regional distribution of CBF. If substituted for an equipotent concentration of a volatile anesthetic agent, nitrous oxide increases CBF. For the normal brain, the resulting cerebral vasodilation can be controlled by hypocapnia or the addition of an intravenous anesthetic. However, volatile agents have no such attenuating effect; CMR and CBF are higher during 1 MAC anesthesia produced by a nitrous oxide-volatile anesthetic combination than that produced only by a volatile anesthetic. This effect is especially deleterious in the actual or potential presence of brain ischemia. Particularly for repeat craniotomy, the potential of nitrous oxide, which is poorly soluble, to diffuse into and hence expand hollow spaces must be remembered as it could cause tension pneumocephalus in patients with intracranial air (repeat neurosurgery or head trauma).
Opioids
Opioids have been associated with short-term increases in ICP, particularly sufentanil or alfentanil. Reflex cerebral vasodilation after decreases in MAP and hence in CPP is the underlying mechanism for the transient increases in ICP, , although a direct modest cerebral vasodilator effect has been demonstrated. This effect demonstrates the sensitivity of intracerebral drug effects to the intracranial and extracranial environment and the importance of maintaining normovolemia for ICP stability. Generally, opioids modestly reduce CMR and do not affect flow-metabolism coupling, autoregulation, or the carbon dioxide sensitivity of the cerebral vessels. Remifentanil has been extensively studied. Its cerebral effects are comparable to those of other opioids, and its use in neuroanesthesia has been validated in clinical trials. ,
Other Drugs
Vasodilating antihypertensive agents such as nitroglycerine, nitroprusside, and nicardipine increase ICP and should be avoided. Cerebral vasodilation may result from a normal autoregulation response or direct arterial vasodilation. For example, sodium nitroprusside increases ICP, but intracarotid injection of nitroprusside does not change CBF. Conversely, verapamil decreases cerebrovascular resistance in humans by inducing direct cerebral vasodilation. Theophylline constricts cerebral vessels but increases CSF production and is a potent central nervous system (CNS) stimulant, raising the risk of convulsions. Most β-adrenergic blockers, especially esmolol, do not interfere with cerebral blood flow or metabolism.
Reducing Intracranial Pressure, Brain Bulk, and Tension
The anesthesiologist possesses a number of instruments to achieve ICP reduction and brain relaxation ( Box 11.2 ), and thus to improve the quality of surgical exposure and to reduce retractor pressure. The effectiveness of these instruments depends on intact intracerebral homeostatic mechanisms.
Prevention
Euvolemia
Sedation, analgesia, anxiolysis
No noxious stimulus applied without sedation and local anesthesia
Head-up position, no compression of the jugular veins, head straight
Osmotic agents: mannitol, hypertonic saline
β-Blockers or clonidine or lidocaine
Steroids, if a tumor is present
Adequate hemodynamics: mean arterial blood pressure, central venous pressure, pulmonary capillary wedge pressure, heart rate
Adequate ventilation: Pao 2 > 100 mmHg, PaCO 2 35 mmHg
Intrathoracic pressure as low as possible
Hyperventilation on demand before induction
Use of intravenous anesthetic agents for induction and maintenance in case of tensed brain
Treatment
Cerebrospinal fluid drainage if ventricular or lumbar catheter in situ
Osmotic agents
Hyperventilation
Augmentation of anesthesia with intravenous anesthetic agents: propofol, thiopentone, etomidate
Muscle relaxants
Venous drainage: head up, no positive end-expiratory pressure, reduction of inspiratory time
Mild controlled hypertension if autoregulation present
Intravenous Anesthetics
Intravenous anesthetics reduce CMR, CBF, and hence CBV and ICP, leading to a diminution of brain bulk, as discussed previously. Cerebral vasoconstriction depends on intact flow-metabolism coupling ( Figs. 11.4 and 11.5 ) and is dose related up to neuronal electrical silence (EEG burst suppression). Like autoregulation, flow-metabolism coupling is impaired by brain contusion and other intracerebral pathologic conditions.

Hyperventilation
Hyperventilation results in hypocapnia and subsequent cerebral vasoconstriction. In the context of intact autoregulation, CBF is roughly linearly related to PaCO 2 between 20 and 70 mmHg. However, the carbon dioxide reactivity of cerebral vessels may be impaired or abolished in the presence of head injury or other intracerebral pathologic conditions, by high inspired concentrations of volatile anesthetics, or, particularly if the vessels are already dilated, by nitrous oxide. The CBF-, CBV-, and ICP-reducing effects of hypocapnia are acute and apparent for less than 24 hours. A typical value to aim for is a PaCO 2 of 30 to 35 mmHg; arterial blood gas analysis rather than end-tidal CO 2 ( etco 2 ) should be used as a controlling variable because of the possibility of large arterioalveolar CO 2 gradients in neurosurgical patients. The effectiveness of hyperventilation (PaCO 2 at 25 ± 2 mmHg) for controlling brain bulk in the patient under either isoflurane or propofol anesthesia has been demonstrated.
The main complication associated with hyperventilation is reduction of CBF, which gives rise to cerebral ischemia. Thus, the anesthesiologist must balance the benefit of brain relaxation against the risk of cerebral hypoperfusion. Other side effects are linear reduction in coronary artery flow, reduced cardiac venous return, hypokalemia, and potentiation of the brain’s response to opioids.
Diuretics and Osmotic agents
Osmotic diuretics such as mannitol and hyperosmotic saline increase blood osmolality acutely, thus reducing brain water content (mainly in healthy brain tissue with an intact blood–brain barrier) and hence brain bulk and ICP. This response improves brain deformability and thereby facilitates surgical exposure. A further beneficial effect is improvement in blood rheology as a result of the reduction in edema of vascular endothelium and erythrocytes (increasing erythrocyte deformability)—the basis of mannitol’s classic “antisludge” effect. A typical regimen is to give 0.5 to 1 g/kg mannitol (150–400 mL 20% mannitol) intravenously, split between a more rapid pre-craniotomy dose and a slower infusion, until brain dissection is complete. The ICP effect is prompt, removes about 90 mL of brain water at peak effect, and lasts for 2 to 3 hours. Theoretically, equiosmolar infusions of hypertonic saline or mannitol should have the same effect for reducing brain water content. One study showed slightly better results with hypertonic saline than mannitol on intraoperative brain relaxation. Normally the aim is to keep osmolality at less than 320 mOsm/kg. Problems with the use of osmotic diuretics include hypernatremia, hypokalemia, and acute hypervolemia, which could be deleterious in patients with congestive heart failure. There is no additional benefit to using loop diuretics such as furosemide, which induces hypovolemia and does not reduce brain water content except that it may limit rebound edema formation. On the contrary, serum saline should be infused to replace urinary losses in order to avoid hypovolemia and maintain blood pressure.
Cerebrospinal Fluid Drainage
CSF drainage is achieved either by intraoperative direct puncture of the lateral ventricle or through a lumbar spinal catheter placed preoperatively. The latter is effective only if there is no caudal block to CSF outflow. Because of the risk of causing acute brain herniation, lumbar CSF drainage should be used cautiously and only when the dura is open. The patient should receive at least mild hyperventilation when CSF is drained. Normally removal of 10–20 mL of CSF is very effective in reducing brain tension. Up to 50 mL can be drained if necessary.
Other Factors
Other factors causing cerebral vasodilation and that can be corrected by the anesthesiologist include hypovolemia and hypoxia. The position of the patient (head down, extreme turning of the neck) also influences brain volume because of impaired venous drainage of the brain. This should be kept in mind when brain swelling is observed without any obvious reason after the dura is opened. Repositioning of the head to avoid excessive rotation and compression of the jugular vein may be the solution.
Vasoconstrictive Cascade
Finally, the anesthesiologist can use the vasoconstrictive cascade by mildly increasing MAP, thus increasing CPP and decreasing CBV and ICP ( Fig. 11.6 ).

General Anesthetic Management
Preoperative Assessment
Determination of anesthetic strategy for a given neurosurgical intervention depends on thorough knowledge of the neurologic and general state of the patient, the planned intervention, and holistic integration of these factors. The patient and the planned intervention should be discussed with the neurosurgeon involved.
Neurologic State of Patient
A major aim in evaluating neurologic status is to estimate how much ICP is raised, the extent of impairment of intracranial compliance and autoregulation, the localization of the tumor and how much homeostatic reserve for ICP and CBF remains before brain ischemia and neurologic impairment occur. The goal is also to assess how much permanent and reversible neurologic damage is already present. Typical pointers to these elements in the patient history, physical examination, and technical examinations are listed in Box 11.3 . The minimum examination should involve a neurologic mini-mental status assessment, comprising the patient’s ability to follow commands, the patient’s degree of orientation, the presence or absence of speech deficit, the pupil symmetry and the Glasgow Coma Scale score. Elucidating what medication the patient is receiving and for how long is important because this medication may also affect intracranial compliance, perfusion, and reserves, as well as modify the pharmacokinetics and dynamics of anesthetic drugs.
History
Seizure (type, frequency, treatment)
Increased intracranial pressure (ICP): headache, nausea, vomiting, blurred vision
Decreased level of consciousness, somnolence
Focal neurologic signs: hemiparesis, sensory deficits, cranial nerve deficits, etc.
Paraneoplastic syndromes, including presence of thrombosis
Physical Evaluation
Mental status
Papilledema (increased ICP)
Signs of Cushing’s response: hypertensive bradycardia
Pupil size, speech deficit, Glasgow Coma Scale score, focal signs
Medication
Steroids
Antiepileptic drugs
Technical Examination (Computed Tomography or Magnetic Resonance Imaging)
Size and location of the tumor: silent or eloquent area, near a major vessel, etc.
Intracranial mass effect: midline shift, decreased size of the ventricles, temporal lobe hernia
Intracranial mass effect: hydrocephalus, cerebrospinal fluid space around brainstem
Others: edema, brainstem involvement, pneumocephalus (repeat craniotomy)
Evaluation of Hydration Status
Fever; infection
Duration of bed rest
Fluid intake
Diuretics
Inappropriate secretion of antidiuretic hormone
Neurologic Working Diagnosis
Tissue type of tumor
The patient’s CT scan or magnetic resonance image should be examined for the size and localization of the tumor and for signs of increased ICP. The latter include effacement of the lateral ventricle by tumor mass, lateral ventricle extension resulting from obstructive hydrocephalus, and midline shift (midline shift > 5 mm). The presence of such signs warns that the ICP-volume curve is close to decompensation (the “knee” of the hyperbolic ICP-volume curve; see Fig. 11.1 ), with small increases in intracranial volume leading to disproportionate ICP increases and brain swelling. The preoperative treatment of brain edema with steroids should not mislead the neuroanesthetist into thinking that the patient is no longer at risk for perioperative intracranial hypertension. Any patient who has presented with symptoms and signs of elevated ICP should be regarded as being at risk for perioperative intracranial hypertension even if the presenting clinical tableau is no longer present.
General State of Patient
Cardiovascular and respiratory functions are important because brain perfusion and oxygenation ultimately depend on them; their function should therefore be optimized preoperatively. Some intracranial pathologic conditions alter cardiovascular function (e.g., effects of raised ICP on cardiac conduction). Supratentorial surgery (particularly for meningiomas, metastasis) can be associated with significant blood loss, and hypovolemia and hypotension have detrimental effects in the neurosurgical context. The neuroanesthetist should note that both hyperventilation—often used to control ICP, CBF, CBV, and brain tension—and the operative position make additional demands on the respiratory and cardiovascular systems. Finally, especially in neurosurgery for metastases, the primary tumor can itself impair cardiorespiratory function (e.g., 40% of brain metastases originate from the lung ) as can anticancer chemotherapy or radiotherapy. Examples are cardiomyopathy and doxorubicin (Adriamycin) or cyclophosphamide (Cytoxan) therapy and inhibition of plasma cholinesterase activity.
Further problems associated with malignant tumors include coagulation disorders, which are associated with an increased risk of thromboembolism, as high as 21% in the first year after surgery. Thus, despite the risk of bleeding, low-molecular-weight heparin may be indicated after craniotomy to prevent venous thromboembolism in high-risk patients.
Other systems interacting with neuroanesthesia are the renal system (e.g., diuretics and subsequent changes in plasma electrolytes, diabetes insipidus, decreased fluid intake), the endocrine system (altered by the intracranial disease process, such as pituitary adenoma, or by therapeutic drugs, such as the effect of glucocorticosteroids on hyperglycemia and cerebral ischemia), and the gastrointestinal tract (e.g., mucosal effects of steroids, motility effects of raised ICP). Hypercalcemia should be ruled out when brain tumors are associated with bone metastasis. A thorough history, supplemented by appropriate physical and technical examinations, is important for the elucidation and definition of these problems. It is important to remember that elderly patients (especially those with impaired cardiac and pulmonary function) pose particular challenges for anesthetic and perioperative management in this context.
Planned Operative Intervention
For a planned operative intervention, the important points to clarify include the size and position of the tumor, the tissue diagnosis, the surgical approach, the structures in proximity and the likelihood of their involvement by surgery, and whether the tumor is to be removed radically. Knowing whether the mass to be resected is a tumor, a hematoma (acute or chronic), an abscess, a metastasis, or something else is useful information. The surgical approach determines the positioning of the patient; common approaches to supratentorial masses are either pterional or temporal and frontal craniotomies. In a bifrontal approach, the sagittal venous sinus is traversed, thereby raising the risk of bleeding and venous air embolism.
When the tissue diagnosis is meningioma, the anesthesiologist should anticipate an operation with the goal of complete excision, which is generally curative. Meningiomas can grow quite large, particularly in neurologically silent areas such as the frontal region, because they often grow slowly. They are often in difficult locations because of either surrounding structures or problematic access (e.g., sagittal sinus, optic nerve sheath, clivus, tentorial notch, ventricles, bone invasion). The combination of large size, difficult location, and the desire for radical excision makes for long and technically demanding operations. Such procedures are often accompanied by significant bleeding (from surrounding structures and because meningiomas are often highly vascular) and require maximal reduction of brain tension to facilitate surgical access. Preoperative embolization may reduce intraoperative bleeding during meningioma resection. Intraoperative blood salvage and autotransfusion may limit homologous transfusion in about 15% of patients. In contrast, glioma resections are often easy debulking operations, of simple surgical access and low propensity to stimulate bleeding.
Colloid cysts of the third ventricle and epidermoid tumors arising in the basal cisterns are the most common nonpituitary supratentorial lesions. Colloid cysts of the third ventricle may be accompanied by obstructive hydrocephalus and thus high ICP at anesthesia induction. The relatively deep location of colloid cysts, epidermoid tumors of the basal cisterns, and pituitary tumors (if operated transcranially) make the provision of excellent brain relaxation for their exposure at the skull base the major anesthesiologic challenge during resection. Transsphenoidal resection of a pituitary adenoma is an essentially extracranial operation.
Determination of Anesthetic Strategy
After consideration of the preceding factors, the following issues are addressed:
Vascular access: Consider risk of bleeding and venous air embolism, need for hemodynamic and metabolic monitoring, and requirements for infusion of anesthesia (TCI), vasoactive and other substances.
Fluid therapy: Aim for normovolemia and normotension, avoid hypo-osmolar fluids (lactated Ringer’s solution), and avoid glucose-containing solutions in order to prevent hyperglycemia, which exacerbates ischemic brain injury.
Anesthetic regimen: Use (1) volatile agent-based anesthesia for “simple” procedures with low risk of ICP problems, ischemia, and need for brain relaxation and (2) total intravenous anesthesia for more complex procedures with anticipation of ICP problems, significant risk of cerebral ischemia, and need for excellent brain relaxation.
Ventilatory regimen: Aim for normocapnia or mild hypocapnia, mild hyperoxia, and low intrathoracic pressures (to improve cerebral venous return).
Extracranial monitoring: Assess cardiovascular and renal function (anticipate the management of venous air embolism). Monitor bleeding with point-of-care devices such as ROTEM®. TEG® and Multiplate®.
Intracranial monitoring: Check general intracranial environment versus specific functions or pathways, for example, neurophysiologic (EEG, evoked potentials), metabolic (jugular venous bulb oxygenation, transcranial oximetry), and functional (transcranial Doppler ultrasonography).
Special techniques : Take into account surgical needs that may modify anesthetic management (brain stimulation and use of muscle relaxants, for example).
Preoperative Preparation
Premedication
Sedation carries the risk of hypercapnia, hypoxemia, and partial upper airway obstruction, all of which are detrimental in the context of increased ICP. However, avoiding stress (increased CMR, CBF) and hypertension (increased CBF, possibly vasogenic edema with impaired autoregulation) is also desirable. Thus analgesia and sedation (e.g., midazolam 0.5 to 2 mg or other benzodiazepines and/or fentanyl 25 to 100 μg or sufentanil 5 to 20 μg) may be provided during the placement of preoperative vascular access and monitoring devices by small, titrated, and intravenous increments under the direct and continuing control and observation of the anesthesiologist. The patient must never be left unsupervised in this context; respiratory support should be provided as necessary. However, in patients with tumors with no clinical or other signs of increased ICP (no shift, etc.), a small dose of benzodiazepine can help decrease the level of anxiety while keeping in mind to use medications allowing proper postoperative assessment. Small doses of benzodiazepines or narcotics can unmask or worsen a preexisting compensated neurologic deficit. This event may be difficult to distinguish from rapid worsening of mass effect and intracranial hypertension.
Steroids should be continued on the morning of the operation (methylprednisolone or dexamethasone). Histamine (H 2 ) blockers and gastric prokinetic agents should be considered to counteract the reduced gastric emptying and greater acid secretion associated with increased ICP and steroid therapy, particularly in patients with cranial nerve (IX, X) palsies (impairment or absence of gag reflexes). Other regular medication, particularly anticonvulsants, as well as antihypertensive and other cardiac medications, should be continued, although drug interactions may occur with phenytoin. Perioperative fluctuations in antiepileptic medications may occur, contributing to perioperative development of seizures. Monitoring of plasma levels or temporary augmentation of dosage of such medications may be necessary.
Vascular Access
Two large-bore peripheral intravenous lines are usually placed for a full craniotomy; one line should suffice for stereotactic biopsy. Central venous access, currently less systematically inserted, is indicated if a clinically significant risk of venous air embolism exists, if substantial bleeding is anticipated (e.g., a large vascular tumor, proximity to major arteries or venous sinuses, or extensive bone resection), if major cardiovascular compromise is evident or suspected, and if vasoactive drugs are to be infused continuously. We recommend the internal jugular approach with a meticulous cannulation technique and minimization of head-down positioning and neck rotation. Such positioning and neck rotation, together with the inevitable element of patient discomfort, can increase ICP. Thus in a stable patient, consideration can be given to putting these lines in once the patient is asleep. If the central line is placed for the management of venous air embolism, its position must be carefully controlled radiographically (tip at transition between vena cava and right atrium) or with the use of an electrocardiography lead.
Neuroanesthesia for full craniotomy with arterial cannulation is recommended, because of the need for very tight monitoring and control of CPP (obtainable by transducing arterial pressure at the mid-ear level of the circle of Willis and using the formula CPP = MAP − ICP). After the dura is opened, ICP equals the atmospheric pressure, so CPP equals MAP. In addition, frequent blood sampling is necessary for measurements of PaCO 2 , particularly if the patient is hyperventilated, is elderly, or has chronic obstructive pulmonary disease, and for plasma glucose, potassium, osmolality, and other measurements. Monitoring of etco 2 is no substitute for PaCO 2 measurement because the two often correlate poorly, especially with impaired ventilation–perfusion matching as occurs in chronic obstructive pulmonary disease, in elderly patients, or with long procedures.
The monitoring of jugular venous bulb oxygen saturation via a catheter placed by retrograde cannulation of the internal jugular vein, permits continuous monitoring of cerebral oxygen extraction and hemoglobin oxygen saturation in jugular venous bulb blood (Sjvo 2 ). Under the assumption that CMR is constant, or through observation of its alteration by EEG monitoring, conclusions can be drawn about the global adequacy of cerebral perfusion ( Fig. 11.7 ).

Monitoring
As already noted, close hemodynamic monitoring is fundamental during neurosurgery. This includes beat-to-beat monitoring of arterial blood pressure and the electrocardiogram for the diagnosis of myocardial ischemia and arrhythmias. Pulse oximetry (for the detection of systemic hypoxia), etco 2 (as a trend monitor for PaCO 2 and to help in the detection of venous air metabolism), and temperature monitoring (eg, esophageal or urinary bladder) represent standard monitoring. A urinary catheter is placed to monitor urine output.
The occurrence of air embolism is best detected by transesophageal echocardiography or precordial Doppler ultrasonography, the most sensitive monitor (together with transesophageal echocardiography) for air bubbles in the venous circulation. If myorelaxants are necessary during the operation, neuromuscular block should be monitored. However, neuromuscular transmission should not be monitored on hemiplegic extremities because the greater acetylcholine receptor density of lower motor neuron units innervated by dysfunctional or nonfunctional upper motor neurons leads to resistance to nondepolarizing myorelaxants. If myorelaxant dosing is based on peripheral nerve stimulation of a hemiplegic extremity, overdosing of normal neuromuscular units will result. In this context hemiparesis is probably not associated with hyperkalemia as appears in paraplegic or burn patients, and the use of succinylcholine is therefore not contraindicated from this point of view. Because general anesthesia and steroid therapy both raise blood glucose levels and because brain retraction is often associated with at least some focal cerebral ischemia, blood glucose levels should be monitored regularly; hyperglycemia worsens neuronal damage during ischemia. In this context, monitoring plasma electrolytes (particularly potassium) and osmolality (particularly if mannitol or hypertonic saline is used) would also appear to be prudent, as would hemoglobin and hematocrit determinations in the context of bleeding.
Particular attention must be paid to the monitoring of coagulation and hemostasis disorders. Primary or metastatic brain tumors may result in vascular perturbations that are thought to contribute to the progression of underlying diseases, such as thrombosis and hemorrhage. Endothelial injury, ischemia and secondary inflammatory reactions trigger the release of brain thromboplastins, thrombin, and iron while degradation products of lysed red blood cells will lead to hemostatic perturbations.
Increased expression of tissue factor, for example, may be related to astrocytic tumors, while specific brain tumors have a direct effect on fibrinolysis or enhance plasmatic coagulation. Moreover, the use of antiepileptic drugs has been related to hemostatic disorders, such as platelet dysfunction, hypofibrinogenemia or lowered factor XIII.
In addition to the routine coagulation parameters, point-of care (POC) viscoelastic assays (ROTEM® or TEG®) or whole-blood impedance aggregometry (Multiplate®) can provide an overall understanding of the coagulation status and platelet function. They are performed in whole blood and provide clinically relevant data, such as the speed and quality of clot formation, clot firmness, the presence of hyperfibrinolysis or the effect of aspirin, clopidrogel and GP IIb/IIIA receptor blockers. First reports of their use in neurosurgical cases are published. The availability of rapid POC tests can guide intraoperative therapeutic management and allow goal-directed hemostatic therapy in neurosurgical patients.
Monitoring of the intracranial environment or cerebral function is increasingly practiced during neurosurgery. For some operations, monitoring of evoked potentials is helpful in observing the intactness of specific central nervous pathways. The surgical treatment of tumors located near eloquent brain areas carries a high risk of worsening neurologic deficits. Intraoperative electrostimulation (IES) has been developed to optimize the benefit–risk ratio of surgery. Motor mapping with the patient under general anesthesia or conscious sedation is being increasingly used to localize motor cortex adequately and improve the quality of surgical tumor or of an epileptic zone removal (see Chapter 17 ). Compared with general anesthesia, conscious sedation improved the chance of achieving successful stimulation.
Preoperative ICP monitoring for elective supratentorial tumor surgery is rarely used today because of the impact of corticosteroids on preoperative ICP reduction and the ability of modern anesthetic techniques to control ICP during induction. This does not apply to neurotraumatology, in which ICP monitoring is vital for therapy from the patient’s entry in the emergency department onward. With the advent of relatively safe and simple-to-use catheter-tip ICP monitoring, there is, however, a trend toward more use of postoperative ICP monitoring, particularly for patients at risk (especially for removal of large tumors with extensive surrounding edema or for emergency surgery in patients with intracranial hypertension and altered consciousness). Intracranial hypertension develops in up to 20% of patients in the immediate postoperative period from brain swelling or hematoma formation, and such patients benefit from prompt therapeutic intervention. Postoperative ICP monitoring may also help with the differential diagnosis in patients who do not emerge from anesthesia after operation. For all of these uses, looking at the shape of the ICP curve is important to ensure that the pressures displayed are reliable.
In some specific cases, if lumbar CSF drainage is used, it can provide a reflection of ICP as long as the CSF pathways are not blocked. This approach can be tested by determining whether compression of the jugular veins increases lumbar CSF pressure (Queckenstedt maneuver). The lumbar CSF pressure can then be used to provide information to the surgeon and anesthesiologist about the influence of positioning, anesthesia, and surgery on potential brain perfusion pressure.
Transcranial Doppler ultrasonography (TCD) is being increasingly used in anesthesia and intensive care for monitoring blood flow velocity (FV) (see Chapter 7 ). TCD allows estimation of pressure autoregulation and CO 2 reactivity. In addition, TCD is the only convenient noninvasive method both to detect intracranial complications that lead to increased ICP and to assess cerebral perfusion in anesthetized patients ( Fig. 11.8 ). During emergence from anesthesia, FV monitoring can detect cerebral hyperemia. Although the implications of this physiologic change are not clear, cerebral hemorrhage after severe cerebral hyperemia has been reported. Intraoperative Doppler monitoring in the surgical field, by means of a microvascular ultrasonic flow probe, has been used mostly in aneurysm surgery. It may be used in tumor surgery to assess vessel patency during difficult dissection from the tumor.

Induction of Anesthesia
Goals and Drugs
The major factors to be considered for anesthesia induction for elective supratentorial neurosurgery are avoidance of secondary brain injuries. Therefore, ventilatory control (avoidance of hypercapnia and hypoxemia), sympathetic and thus blood pressure control (ie, adequate depth of anesthesia and antinociception to prevent CNS arousal), and prevention of cranial venous outflow obstruction (head positioning) are essential. Attention to these details improves the patient’s intracranial pressure–volume curve status, ensures the adequacy of cerebral perfusion, helps prevent untoward increases in ICP, and decreases brain perfusion pressure. A typical scheme for achieving these goals is detailed in Box 11.4 , with propofol or thiopental given as a “starter,” and an opioid, together with gentle hyperventilation, administered before intubation. For induction in more frail or elderly patients, etomidate (0.2 to 0.4 mg/kg) may be used instead of propofol.
- 1.
Adequate anxiolysis in the anesthetic room. Adequate fluid loading (5 to 7 mL/kg of NaCl 0.9%). Electrocardiogram leads in place; capnometer, pulse oximeter, and noninvasive blood pressure monitors. Insertion of intravenous and arterial lines under local anesthesia.
- 2.
Induction of general anesthesia. Fentanyl 1 to 2 μg/kg or sufentanil or remifentanil. Preoxygenation and voluntary hyperventilation propofol 1.25 to 2.5 mg/kg or thiopentone 3 to 6 mg/kg for induction. Nondepolarizing muscle relaxant: vecuronium, rocuronium, or cisatracurium. Controlled ventilation at PaCO 2 of 35 mmHg propofol 50 to 150 μg/kg/min or isoflurane 0.5% to 1.5% (or sevoflurane or desflurane) for maintenance and fentanyl (or alfentanil, sufentanil, or remifentanil) 1 to 2 μg/kg/h (or bolus) for analgesia.
- 3.
Intubation.
- 4.
Local anesthesia or intravenous remifentanil 0.5 to 1 μg/kg for skull-pin head-holder placement and skin incision.
- 5.
Adequate head-up positioning; no compression of the jugular veins.
- 6.
Brain relaxation. Mannitol 0.5 to 0.75 g/kg if needed. Insertion of a lumbar drain if needed. Normovolemia with the use of NaCl 0.9% or starch 6%—no lactated Ringer’s solution.
Fentanyl may be replaced by alfentanil (5 to 10 μg/kg followed by infusion at 5 to 10 μg/kg/h), by sufentanil (0.5 to 1.5 μg/kg followed by infusion at 0.1 to 0.3 μg/kg/h) for smoother hemodynamic control, or by remifentanil (0.25 to 0.5 μg/kg followed by infusion at 0.1 to 0.2 μg/kg/h) for rapid awakening and early neurologic assessment independent of the duration of anesthesia.
Muscle Relaxants
Modern nondepolarizing myorelaxants have minimal effects on intracerebral hemodynamics. It is thought that the use of succinylcholine should be reserved for patients with possible intubation difficulties or when rapid-sequence induction is absolutely unavoidable. Succinylcholine can cause transient increases in CMR, CBF, and ICP, although such increases usually can be controlled by hyperventilation or deepened anesthesia and are of consequence mainly in patients who have precariously elevated ICP.
We strongly recommend avoiding longer-acting myorelaxants, such as pancuronium, and prefer the use of middle- to short-acting myorelaxants, such as vecuronium, cisatracurium, mivacurium, and rocuronium. Our recommendation is based on the fact that neurosurgical patients are particularly susceptible to the effects of myorelaxant hangover (difficult to detect with manual assessment of peripheral nerve stimulation). In this context, the interaction (need for up to 50% to 60% higher doses) between long-term phenytoin or carbamazepine treatment (> 7 days) and pancuronium, vecuronium, atracurium, or cisatracurium should be noted, as should the need to monitor neuromuscular transmission on nonhemiplegic extremities (as discussed previously). The anesthesiologist should keep in mind, however, that immobility of the patient must be guaranteed during the procedure.
Patient Positioning
Pin holder application is a maximal nociceptive stimulus. It must be adequately blocked by deepening of analgesia (bolus of remifentanil 0.25 to 1 μg/kg) or anesthesia (e.g., intravenous bolus of propofol 0.5 mg/kg), preferably in conjunction with local anesthetic infiltration of the pin site to prevent undesirable CNS arousal and hemodynamic activation. Alternatively, hemodynamic control can be achieved with antihypertensive agents such as esmolol (1 mg/kg) and labetalol (0.5 to 1 mg/kg). Scalp blockade, ideally before pinning, improves intraoperative hemodynamic stability and provides some degree of postoperative analgesia. Pin insertion can be associated in very rare cases with venous air embolism in head-up positioned patients .
Patient positioning must be closely surveyed by both the anesthesiologist and the surgeon, with extreme positioning being avoided. Careful attention should be paid to padding or fixing of regions susceptible to injury by pressure, abrasion, or movement, such as falling extremities. A mild head-up position helps venous drainage. Severe lateral extension or flexion of the head on the neck should be prevented (there should be at least two fingers’ space between chin and nearest bone) to avoid endotracheal tube kinking, postoperative airway swelling and compromise, and impairment of cerebral venous drainage (brain swelling). The knees should be mildly flexed to avoid lumbosacral injury. If the head is turned laterally (e.g., for pterional or frontotemporal craniotomy), the contralateral shoulder should be elevated (with a wedge or roll) to prevent brachial plexus stretch injury. The lateral and sitting positions have their own specific positioning precautions. The endotracheal tube must be fixed and packed securely to prevent accidental extubation or abrasions resulting from movement and must be accessible intraoperatively (note: there is increased dead space if extension tubing is used distal to the Y-piece). Finally, the eyes should be taped closed to prevent corneal damage from exposure or irrigation with antiseptic or other fluids.
Maintenance of Anesthesia
Goals
The main anesthetic aims during supratentorial surgery are (1) control of brain tension via control of CBF and CMR (the so-called chemical brain retractor concept [ Box 11.5 ]) and (2) neuroprotection through maintenance of an optimal intracranial environment. The first goal depends on the prevention of CNS arousal; it is achieved through good depth of anesthesia and antinociception ( Fig. 11.9 ), antiepileptic prophylaxis, as well as control of the consequences of CNS arousal should it occur (with antihypertensives, sympatholytics). The second goal depends on maintaining a good match between cerebral substrate demand and supply, as well as attempts at specific neuroprotection if mismatch occurs (note: ischemia occurs under the retractor in 5% to 10% of patients). Some anesthesiologists use modest passive hypothermia (35 °C) to provide neuroprotection, on the basis of the abundant experimental literature demonstrating its efficacy after brain injury. However, clinical studies have not demonstrated any beneficial effect of hypothermia in neurosurgical patients. In addition, hypothermia impairs platelet function and the coagulation cascade. Consequently, even mild hypothermia (< 1 °C) may increase blood loss and the risk for transfusion. Although a higher risk of cerebral bleeding due to hypothermia has not been demonstrated during neurosurgery, this theoretical effect should be considered in the risk–benefit analysis of inducing hypothermia. Other complications of hypothermia are surgical wound infection, adverse myocardial outcomes, prolonged recovery, and shivering. Thus, normothermia should be a major goal of neuroanesthetic management. Hypothermia should be considered only in cases with a major risk of cerebral ischemic injury.
Mild hyperosmolality (use NaCl 0.9% [304 mOsm/kg] as baseline infusion; give 20% mannitol [1245 mOsm/kg] 0.5 to 0.75 g/kg or hypertonic saline [7.5%, 2498 mOsm/kg] 2 to 4 mL/kg before bone flap removal)
Intravenous anesthetic agent (propofol), adequate depth of anesthesia
Mild hyperventilation, mild hyperoxygenation
Mild controlled hypertension: mean arterial blood pressure maintained around 100 mmHg in order to decrease cerebral blood volume and intracranial pressure
Normovolemia; no vasodilators
Mild hyperoxia
Together with:
- •
Head-up positioning with unimpeded cerebral venous drainage; no compression of the jugular veins
- •
Minimal positive end-expiratory pressure
- •
Adequate anesthetic depth or muscle relaxant to prevent bucking on ventilator
- •
Lumbar drainage
- •
Avoidance of brain retractors

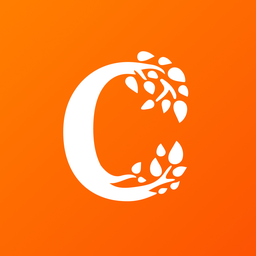
Full access? Get Clinical Tree
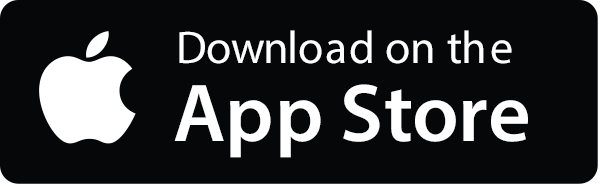
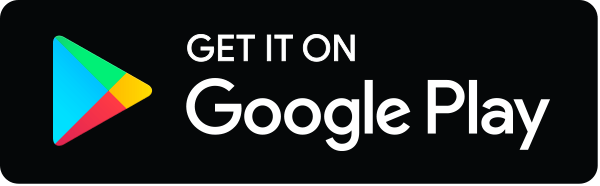
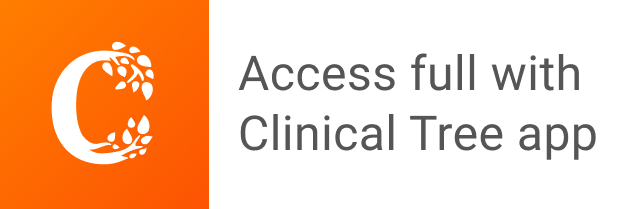