Chapter 79 Structure and Function of Hematopoietic Organs
The hematopoietic system responds quickly to changes in oxygen tension, bleeding, or infection. When stressed, as in a very ill child, hematopoiesis and blood cell function may be insufficient. The extensive use of red blood cell (RBC) or platelet transfusions, and antimicrobials support the importance of the blood system as a vital organ affected in the pediatric intensive care unit setting. This chapter reviews the anatomy and physiology of the hematopoietic system to provide a basis for understanding the repercussions of primary hematological abnormalities, as well as hematopoietic manifestations of nonhematological diseases. Aspects that are of practical importance to the intensivist are emphasized. The structure and function of the coagulation system are discussed in Chapters 81 and 82 and that of the immune system (particularly B and T lymphocytes and macrophages) in Chapter 90.
Structure and Function of the Bone Marrow
During embryogenesis and fetal development, hematopoiesis shifts from the yolk sac to the liver and, after the twentieth week of gestation, to the bone marrow.1 Although hepatic erythropoiesis may persist for several weeks after birth, in the term infant hematopoiesis takes place almost entirely in the bone marrow. A defining feature of hematopoietic stem cells is their ability to home to the bone marrow. This homing occurs via chemoattractants such as stromal cell‑derived factor-1 and its cognate chemokine receptor on the stem cell, as well as interaction between a variety of stem cell adhesion molecules and their ligands on stroma and endothelial cells. The hematopoietic stem cell may be identified by the presence of cell surface markers, such as CD34 or by properties to exclude Hoeschst dye 33342, via a multidrug transporter.2
Grossly, two types of bone marrow can be recognized in normal individuals: yellow marrow, so called because of the predominance of fat cells, and red marrow, in which blood cells predominate. White marrow, consisting predominantly of stromal cells and intercellular matrix, may result from atrophy or starvation. Red marrow proportionately is a much greater component of body weight and volume in the infant than in the adult (Figure 79-1).3 Early in life, it is contained in the medullary cavities of the long bones, which gradually fill with fat such that by late puberty, the adult distribution of hematopoiesis (sternum, pelvis, vertebrae, cranium, ribs, epiphyses of long bones) is achieved. That the degree of hematopoiesis in a given bone may vary with age is an important consideration in selecting a site for bone marrow aspiration or biopsy; for example, although the anterior and posterior iliac crests can be used at any age, the tibia can be used only until the age of 2 years. In disease states characterized by excessive destruction of blood cells, such as some severe hemolytic anemias, hematopoiesis may increase twofold to eightfold. Active sites of hematopoiesis may expand, and extramedullary hematopoiesis may be found, particularly in the liver and spleen.
Microscopically, the marrow is a network of vascular channels (sinuses) separating islands of fat, hematopoietic cells, and rare osteoblasts and osteoclasts (which are important for bone remodeling).4,5 The vasculature and cells are joined by a reticulin (fiber) network or scaffolding. By light microscopy, bone marrow aspirate specimens demonstrate hematopoietic elements; however, a bone marrow biopsy provides a more accurate measure of cellularity. Reticulin can be seen by light microscopy when special histochemical stains are used.
The blood vessels that feed the marrow are branches of those that feed the surrounding bone.6 Large central arteries run longitudinally within the marrow and send radial branches that penetrate the endosteum and form capillaries in the Haversian and Volkmann’s canals of the bony cortex.7 These capillary systems drain into the bone marrow sinuses, which in turn drain into a central sinus or vein. Because the marrow circulation interconnects with the general circulation in this fashion, fluids and medication injected in bone marrow are absorbed as rapidly as through intravenous routes. Unlike peripheral veins, intramedullary vessels supported by their bony shell do not collapse in shock; therefore, intraosseous (either tibial or iliac crest) infusion is appropriate when standard intravenous access is not available.8 It is also noteworthy that the interconnection between the marrow and general circulation provides the mechanism by which bone marrow may embolize to the lung after osseous trauma or fracture. This has not been demonstrated to be of clinical significance in the case of intraosseous infusion.9
The concept of the stem cell has expanded recently,10 and its ex vivo manipulation has raised much interest by a wide group of physicians and scientists studying nonhematologic systems. One essential feature of the stem cell is its plasticity.11 Provocative studies have shown that stem cells isolated from the bone marrow can be driven to differentiate to muscle, liver, cardiac, or neuronal tissue. This raises the possibility that the marrow may be a convenient and ethically less challenging source of stem cells for stem cell engineering and tissue replacement. Although the clinical applications to nonhematologic tissues remain distant, the use of hematopoietic stem cells to replace a diseased marrow is a common practice (i.e., stem cell transplantation). Alternative sources of hematopoietic stem cells have been found peripheral blood following growth factor−induced mobilization or from placental cord blood.
Hematopoiesis
Cells within the hematopoietic island include the RBCs, granulocytes (neutrophils, eosinophils, basophils), monocytes and macrophages, platelets, lymphocytes, and their precursors. The earliest precursors, or stem cells, are thought to look like small lymphocytes and are not usually distinguishable from them by microscopy. Their existence was best confirmed by in vitro culture assays in which nucleated cells from bone marrow aspirate specimens plated onto tissue culture dishes layered with methylcellulose generate colonies (aggregates of cells) of one or more lineages. Now, immunophenotyping provides rapid identification of cell lineage and stage of development.12 The first morphologically identifiable precursor cells are the proerythroblast, myeloblast, monoblast, megakaryoblast, and lymphoblast. These committed precursors and their terminally differentiated counterparts sit within the hematopoietic islands. Megakaryocytes (which make up <1% of hematopoietic cells) generally are located next to marrow sinusoids and shed platelets (fragments of megakaryocyte cytoplasm) directly into the lumen. Erythroblasts also are produced near the walls of the vascular sinuses in clusters with macrophages called erythroblast islets. As the erythroblasts develop, they extrude their nuclei, which are phagocytosed by the macrophages. In contrast, granulocytes (most numerous of the hematopoietic cells), monocytes, and lymphocytes are produced throughout the marrow away from vascular sinuses. The mature white blood cells (WBCs) are motile and migrate to the sinuses.
Within the peripheral circulation, the number of cells of each type is maintained in a narrow range in the normal individual. Adults and post-pubertal adolescents have approximately 5000 granulocytes, 2000 lymphocytes, 500 monocytes, 5 × 106 RBCs, and 150,000 to 300,000 platelets per microliter of whole blood. Age-dependent values for younger children are shown in Table 79-1.13 To a lesser extent, values also are a function of race and sex, so that African Americans (especially males) normally may have granulocyte counts less than 1500/μL. Normative values for Latinos are less clear, but have been reported to be closer to those of Caucasians.14 As summarized elsewhere, under normal conditions the rate of production of each cell type equals the rate of destruction. Because the life span of mature RBCs in adults is 100 to 120 days, 5 × 104 RBCs/μL are produced daily. The average platelet life span is 7 to 10 days so that approximately 2 × 104 platelets/μL are produced daily. With less than a 12-hour life span, granulocytes production occurs at a rate of 104 cells/μL. The very slow rate of production of lymphocytes reflects their long life span, measured in years.
The mechanisms that regulate this steady state are incompletely understood. However, evidence strongly suggests the existence of a pluripotent stem cell that is capable of self-renewal, from which progenitor cells committed to hematopoiesis (RBCs, granulocytes, megakaryocytes, and monocytes) and to lymphopoiesis develop (Figure 79-2).13 The “trilineage myeloid” stem cell has been designated colony forming unit-stem (CFU-S) on the basis of bone marrow culture assays and experiments in which the spleens of lethally irradiated mice infused with donor marrow cells are found to contain colonies each consisting of precursors of RBCs, granulocytes, monocytes, and megakaryocytes.16 The existence of CFU-S in human beings is further deduced from chromosomal studies in myeloproliferative disorders. Lymphoid development appears to arise from a separate progenitor. Although the CFU-S is found predominantly in the bone marrow, there probably are small numbers of circulating pluripotent stem cells because marrow of lethally irradiated animals can be reconstituted by using peripheral blood.3
The numbers of committed progenitor cells that differentiate in any time period is dependent on feedback from humoral regulators that are produced within the marrow microenvironment and by extramedullary sources, including T cells, macrophages, endothelial cells, and fibroblasts. These hematopoietic growth factors are cytokines, known by a variety of names. Many of the cytokines have overlapping functions. However, gene targeting in the mouse (“knockout mouse”) of cytokines or their receptors has identified the essential nonredundant functions for several hematopoietic growth factors. Mice deficient in erythropoietin (Epo), thrombopoietin (Tpo), and granulocyte colony-stimulating factor (G-CSF) suffer from severe anemia, thrombocytopenia, or neutropenia, respectively.
Although the list of cytokines and small molecules that regulate hematopoiesis continues to grow, clinical application has been chiefly limited to Epo and G-CSF. Chemical modification of Epo and G-CSF has resulted in two longer-acting forms, darbepoetin alpha, and pegfilgrastim, respectively. Their primary advantage is longer half-lives. Recently, two platelet-stimulating agents, romiplostim and eltrombopag, have been Food and Drug Administration−approved for use in adults with chronic immune thrombocytopenic purpura (ITP).15 Characteristics of specific hematopoietic growth factors, discussed in the following sections, are summarized in Table 79-2.
Table 79–2 Recombinant Hematopoietic Growth Factors
CSF | Target | Clinically Available |
---|---|---|
SCF | HSC, mast cells | No |
IL-3 | HSC | No |
Epo | Erythroid progenitors | Yes |
G-CSF | Granulocytes and their precursors | Yes |
GM-CSF | Phagocytes and their precursors, dendritic cells | Yes |
M-CSF | Monocytes and their precursors | No |
Tpo∗ | Megakaryocytes | Yes∗ |
IL-11 | Megakaryocytes | Yes |
Epo, Erythropoietin; G-CSF, granulocyte colony-stimulating factor; GM-CSF, granulocyte-macrophage colony-stimulating factor; HSC, hematopoietic stem cells; IL, interleukin; M-CSF, macrophage colony-stimulating factor; SCF, stem cell factor; Tpo, thrombopoietin.
∗ Thrombopoietin-like peptides have been approved.
Erythropoiesis
On its way toward RBC maturation (see Figure 79-2), the CFU-S sequentially differentiates into burst-forming units-erythroid (BFU-E) and colony forming units-erythroid (CFU-E), which are identifiable experimentally on the basis of growth characteristics in culture. These phases of development, which involve amplification of cell number, are extensively reviewed elsewhere.16 As noted earlier, the proerythroblast is the earliest morphologically identifiable precursor and presumably is the successor to the CFU-E. The subsequent sequence of RBC production normally takes 3 to 4 days in the marrow and involves multiple cell divisions with increasing differentiation, characterized chiefly by globin messenger RNA, cytoplasmic synthesis of hemoglobin, and ultimately extrusion of the RBC nucleus. The enucleated cell is large and, because it contains residual RNA, stains deeply by the Wright-Giemsa technique (i.e., polychromatophilic macrocyte). Normally at this stage the erythrocyte is released into the circulation where it can be demonstrated as a reticulocyte. In newborns younger that 1 week of age, reticulocytes in the blood can comprise more than 5% of the total RBCs. At any older age, the normal reticulocyte count is less than 2%. From this uncorrected reticulocyte count, the absolute reticulocyte count can be calculated by multiplying by the RBC count. The normal absolute reticulocyte count would be 0.02 to 5 × 106 RBC/μL. Alternatively, a corrected reticulocyte is used: multiplying the reticulocyte percentage by the observed hematocrit divided by the normal hematocrit. If the corrected reticulocyte count is less than 1%, one must suspect bone marrow failure or insufficiency. Because the reticulocytes lose their RNA within 24 to 30 hours, their quantitation provides a rough estimate of the rate of erythropoiesis during the past 24 hours. This can be more accurately measured by ferrokinetic studies, which are not readily available. The proportion of erythroid precursors in a bone marrow aspirate also provides a more convenient estimate of total erythropoiesis that is valid if a cellular specimen is obtained and if granulopoiesis is normal. In the older child or adult, erythroid precursors normally are one third as plentiful as myeloid precursors (i.e., the myeloid/erythroid ratio is about 3:1). Approximately 10% of erythroid precursors do not produce circulating RBCs (ineffective erythropoiesis).
Maturation of RBC precursors is regulated by a number of humoral and nutritional factors. Epo appears to act predominantly by increasing proliferation of CFU-E (see Table 79-2 and Figure 79-2). Its production is stimulated by hypoxemia or acute hemorrhage. During fetal development, it is mainly produced in the liver, but this site shifts later to the juxtamedullary region in the kidneys. Humoral factors less well characterized than Epo that are derived from multiple sources (spleen cells, peripheral blood monocytes, and mononuclear bone marrow cells) appear to act at an earlier stage of differentiation to amplify the number of progenitors committed to Epo responsiveness. Among these are burst-promoting activity, which enhances production and proliferation of BFU-E. Within the marrow, normal RBC maturation requires both folate and vitamin B12; a deficiency of either results in abnormal nucleic acid synthesis and the production of an abnormal precursor, the megaloblast. Iron is required for hemoglobin synthesis, and deficiency results in poorly hemoglobinized small (hypochromic, microcytic) RBCs.
Once in the peripheral blood, the life span of the normal RBC in the adult or older child is 100 to 120 days. In the term newborn the RBC life span is about 60 days, which grows progressively shorter with increasing prematurity.14 Presumably these differences in age-dependent RBC longevity reflect differences in membrane stability and oxidative metabolism. Removal of RBCs from the circulation is not a random process. Senescent RBCs are removed selectively from the circulation by the macrophages of the reticuloendothelial system. Although this is primarily a function of the spleen (see the following section), asplenic patients with normal RBCs accomplish this process in the liver and other sites and do not exhibit an increased RBC life span. In subjects with hemolytic anemias who undergo therapeutic splenectomy, the red cell life span increases, but not to normal levels.
The primary function of the circulating RBC is to carry O2 from the lungs to the tissues. Hemoglobin (Hb) must be packaged within the RBC membrane to prolong its plasma half-life. Interference with the reversible binding of O2 by Hb can occur by several mechanisms, including (1) methemoglobinemia, the inability to maintain ionic iron within the Hb molecule in the reduced state; (2) the presence of abnormal hemoglobins, among which are the methemoglobins which have an abnormal affinity for O2; (3) age- or disease-related differences in the percentage of structurally normal HbF, which has a high affinity for oxygen; and (4) changes in the microenvironment that alter the intracellular concentration of 2,3-diphosphoglycerate (2,3-DPG).17 The oxyhemoglobin interaction also is complicated by the Bohr effect (at a lower pH, Hb binding of O2 with less affinity) independent of 2,3-DPG concentration. Patients with severe acidosis have low concentrations of 2,3-DPG, which results in an oxygen dissociation curve that shifts to the left. However, the in vivo curve may be normally placed because the Bohr effect counterbalances the reduction in red cell 2,3-DPG. If metabolic acidosis is rapidly corrected, the prompt rise in blood pH is reflected in a proportional increase in oxygen affinity (the Bohr effect). However, there is a lag of several hours before the red cell 2,3-DPG increases to normal. During this time, there is a shift to the left in both the in vivo and the in vitro O2 dissociation curves. This phenomenon may compromise tissue oxygenation in patients who have diminished cardiovascular reserves.
Premature infants with respiratory failure may have a left-shifted O2 affinity curve both because of the high levels of HbF and because of an acidosis-induced decrease in 2,3-DPG levels. Exchange transfusions with fresh adult blood have been found to reduce mortality, perhaps by providing blood with “normal” O2 affinity.18 Other reasons for impaired O2 delivery are decreased RBC mass (anemia, which leads to a compensatory increase in 2,3-DPG) and decreased blood flow, either because of vascular anatomical abnormalities or because of increased blood viscosity (e.g., in sickle cell disease). In addition to Hb-bound O2, the oxygen dissolved in plasma (which normally amounts to less than 2% of the total oxygen carried in the blood) increases linearly with increases in PO2. For this reason, very anemic patients, although they have insufficient Hb with which to carry O2, benefit from administration of O2. Extensive research into the design of artificial blood has focused both on “red blood” (repackaged Hb from senescent RBCs or of Hb produced with genetic engineering) and “white blood” or perfluorocarbons (emulsions that dissolve large amounts of O2), but their use is still limited and investigational. For now, RBC transfusions ameliorate anemia acutely, otherwise Epo may be used to elevate Hb over several weeks. Although antibody-mediated Epo-associated pure red cell aplasia is infrequent,19 familiarity with possible Epo toxicity should accompany its use.
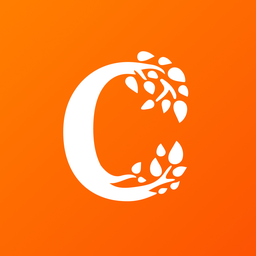
Full access? Get Clinical Tree
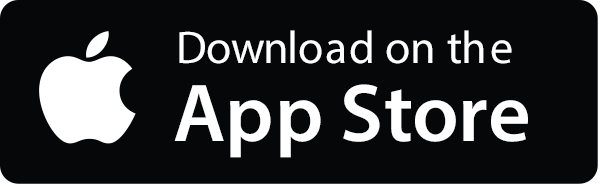
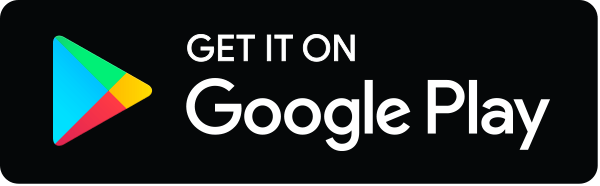