23 Stroke and Brain Protection
John Dunford
II. Pathophysiology of cerebral ischemia
III. Cerebral protection by anesthetic agents
IV. Cerebral protection by nonanesthetic agents
D. Excitatory amino acid modulators
E. Trophic factors, erythropoietin, and cytokines
V. Effective current treatments for cerebral ischemia: Reperfusion and hypothermia
KEY POINTS
1. Stroke is the third leading cause of death and the leading cause of serious, long-term disability in the United States.
2. The neuroanesthesiologist must have a fundamental knowledge of the pathophysiology of neuronal cell death and the appropriate measures, be physiologic or pharmacologic, to prevent and treat ischemic brain injury.
3. Cerebral ischemia results in a rapid uncontrolled increase in intracellular calcium, resulting in excitatory neurotransmitter release, the activation of lipases, proteases, endonucleases, free radical formation, fatty acid production, nitric oxide production, inflammation, and the production caspases. All of these can contribute to neuronal cell death.
4. In contrast to apoptosis (Type I cell death) and necrosis (Type III cell death), autophagy is described as Type II cell death. All three processes can be activated by cerebral ischemia.
5. Anesthetics generally decrease the cerebral metabolic rate by as much as 60% by decreasing neuronal functional activity on the electroencephalogram (EEG) and can increase the supply of oxygen by cerebral vasodilation.
6. Exposure to the brain by anesthetics either immediately or up to a few days prior to the induction of ischemia decreases ischemic brain injury. This is referred to as “preconditioning.”
7. Anesthetics may cause cerebral protection by reduction in ischemia-induced glutamate release, increased GABA-A–mediated hyperpolarization, blockage of postsynaptic glutamate receptors, and increased levels of antiapoptotic proteins.
8. Anesthetic agents have shown cerebral protective effects in vitro and in vivo. The mechanism for cerebral protection by anesthetic agents is most likely multimodal. In vitro and animal studies support the neuroprotective properties of barbiturates, volatile anesthetics, propofol, ketamine, benzodiazepines, and lidocaine. Human clinical trials have been disappointing.
9. Nonanesthetic agents including calcium channel blockers, magnesium, free radical scavengers, excitatory amino acid modulators, erythropoietin, trophic factors, cytokines, statins, and antiapoptotic agents all show potential for cerebral protection. However, with the exception of the use of statins in nonhemorrhagic stroke, more research needs to be performed before these classes of drugs will be found to be clinically useful.
10. Intravenous thrombolytics for ischemic embolic stroke and mild hyoothermia for both spontaneous circulation after out of hospital cardiac arrest and neonates with hypoxic-ishemic encephalopathy are associated with improved outcomes.
I. Introduction
1
A. Stroke is the third leading cause of death and the leading cause of serious, long-term disability in the United States [1,2].
1. Stroke is a dreaded perioperative complication. Cardiopulmonary bypass is associated with a 1.3% to 7.9% risk of focal neurologic deficit [3].
2. There is a 4.8% chance of stroke after the resection of head and neck tumors, and a 5.4% to 6.1% with carotid endarterectomy [4–6].
3. In the United States, the aggregate lifetime cost associated with an estimated 392,344 first-time strokes in 1990 was $40.6 billion dollars [7].
4. Therefore, the ability to provide perioperative cerebral protection both before and after cerebral ischemia would have obvious benefits.
B. Cerebral ischemia can be classified as either global or focal.
1. Global cerebral ischemia results from decreased global cerebral perfusion either from cardiogenic or noncardiogenic causes [8].
2. Focal cerebral ischemia results from small vessel occlusion, arterial emboli, cerebral vasospasm, and direct surgical vascular injury.
3. Focal ischemia is described as having an ischemic “core” and a larger variable zone that is called the “penumbra.”
a. In the core, the lack of blood supply results in rapid neuronal death.
b. In the penumbra, the flow reduction is sufficient to result in an isoelectric EEG, but not severe enough to result in rapid cell loss. If the diminished blood flow to the penumbra continues, then cell death will follow. The opportunity to salvage neurons is greatest in the ischemic penumbra [9].
C. There has been a significant increase recently in our knowledge of the mechanisms of neuronal ischemic cell death particularly in the mechanisms of apoptosis. However, effective therapeutic modalities short of good hemodynamic control, hypothermia, thrombolytic therapy, and perhaps barbiturates have as of yet proved elusive.
II. Pathophysiology of cerebral ischemia
2
A. Histopathologic events
1. Neurons of different areas of the brain vary in their sensitivity to ischemia. This may be secondary to differences in blood supply.
2. In the cerebral cortex, the ischemic injury is often more severe in the parietal and the occipital lobes especially in the depths of the sulci.
3. Within seconds of cerebral ischemia, the brain interstitial space disappears. This is thought to be secondary to cell swelling and sodium influx.
4. At 10 minutes, there is clumping of nuclear material.
5. At 30 minutes, further swelling is seen within the astrocytes, with intracellular swelling of the mitochondria, disappearance of microtubules, and detachment of ribosomes from the endoplasmic reticulum.
6. After 2 hours, the mitochondria show flocculent densities suggesting calcium overload. Lysosomes are not involved at this stage and therefore catastrophic cell death is not yet seen. Lysosomes can be stable for up to 4 hours after an ischemic insult.
7. Therefore, lethal cell injury occurring with ischemia of less than 30 minutes duration is typically histopathologically thought secondary to damage of the mitochondria and ribosomes.
8. On typical histologic cadaveric preparations, the first visible change after cerebral ischemia typically occurs 12 to 24 hours after the ischemic cerebral insult. The affected neurons develop a very eosinophilic cytoplasm and a small pyknotic nucleus sometimes called a “red dead neuron.”
9. This process is often widespread but not often completely uniform in the cerebral cortex with clusters of damaged cells being found next to unaffected cells, even in the same cortical layer.
10. After about 48 to 72 hours, there is softening and disintegration of the infarcted area with pronounced edema of both the infarcted tissue and adjacent tissue. This can cause herniation if the infarct is large enough.
11. Swelling of astrocytes can cause compression of cerebral capillaries. Histologically, there is an initial influx of polymorphonuclear leukocytes (PMNs) followed by macrophages invading and digesting the necrotic tissue. Fibroblasts are only located around blood vessels in the brain; therefore, a scar does not form. Rather, a cystic cavity with fibrillary gliosis is seen.
12. Glial cells respond to hypoxia. Astrocytes swell accumulating glycogen and cytoplasmic filaments. Oligodendrocytes are less affected and microglia participates in the phagocytosis of cellular debris.
3
B. Biochemical events
1. The EEG disappears within 20 seconds of ischemia in a normothermic brain. This is probably secondary to ATP depletion.
2. Within 5 minutes, high energy phosphate levels have been depleted and profound changes in cellular electrolyte balance begins to occur. Sodium and calcium ions enter the cell and potassium leaks out. Water follows the sodium intracellularly resulting in cellular swelling. This is particularly pronounced in the astrocytes.
3. Calcium has an extracellular gradient of 10,000 to 1. This gradient is maintained by the active pumping out of intracellular calcium.
4. Calcium pumps include an ATP-driven membrane pump, exchange for calcium for sodium in the sodium–potassium ATP-driven membrane pump, an ATP-driven sequestration of calcium in the mitochondria, and accumulation of calcium in the mitochondria by an oxidation-dependant mechanism. Three of these four mechanisms require ATP and will fail during ischemia. The resulting increase in mitochondrial calcium causes diminished oxidative phosphorylation resulting in a decrease in ATP production [10,11].
5. Elucidating the exact mechanisms of calcium toxicity and identifying the subcellular compartment playing the most important role in this pathologic process might help in the evaluation of strategies for specific therapeutic interventions.
C. Necrotic cell death
1. There is evidence that excitatory neurotransmitters that are released during ischemia play an important role in the etiology of neuronal ischemic injury. Ischemia increases intracellular levels of calcium and sodium, and the resulting nerve depolarization results in the release of excitatory amino acids, especially glutamate. The glutamate transporter protein pumps glutamate into the extracellular compartment when the neuron is ischemic. Glutamate activates alpha-amino-hydroxy-5-methyl-isoxazole propionic acid (AMPA) and N-methyl-d-aspartate (NMDA) receptors. NMDA receptor activation results in increasing intracellular calcium and the activation of metabotropic receptors via second messenger systems. Also, the glutamate-induced depolarization of the cell results in calcium entry into the cell from voltage-gated calcium channels and from the endoplasmic reticulum. These ionic and biochemical changes that occur in the brain due to increased glutamate are called excitotoxicity.
2. Increased cytoplasmic calcium is deleterious to the neuron. High calcium levels increase the activity of proteases and phospholipases. Phospholipases cause the production of free fatty acids including arachidonic acid from membrane lipids. Free fatty acids damage cell membranes and further decrease the cell’s ability to pump out calcium. The fatty acid, arachidonic acid, causes the release of thromboxane and leukotrienes. Both of these can cause platelet aggregation, clotting, vasospasm, and edema with resultant further compromise of cerebral blood flow.
3. Lactic acidosis is associated with cerebral injury during ischemia. Increased levels of lactic acid result in decreased pH. Decreased pH can damage and inactivate mitochondria while lactic acid degradation of NADH (needed for ATP production) may decrease reperfusion ATP levels [12,13].
4. Free radicals are produced during cerebral ischemia [14,15]. Ischemia in the presence of increased intracellular calcium results in xanthine oxidase production. With reperfusion and the reintroduction of oxygen, in the presence of xanthine oxidase, super oxide anion (O2−), hydroxyl radical (OH−), and free lipid radicals are produced. O2− is also released from the leukotriene-activated neutrophils. The resulting reoxygenation permits active uptake of calcium in an already calcium-enriched mitochondria with reperfusion resulting in extreme calcium overload and free radical release. These free radicals will damage proteins and lipids [16].
5. Neutrophil activation is thought to play a role in cerebral ischemia hours to days after the initial ischemic event [17]. PMNs have been implicated as a significant cause of pathology in cerebral ischemia. This is especially true with reperfusion injury. Their acute activation may be responsible for many of the effects of cerebral ischemia including loss of capillary integrity and loss of cerebral ultrastructure with cerebral reperfusion [18,19]. Activated polymorphonuclear cells can generate lots of hydrogen peroxide. This hydrogen peroxide along with myeloperoxide, also from activated PMNs, reacts with halides such as chloride and bromide to produce hypohalous acids. Chloride is the halide with the highest concentration. Therefore the molecule most produced by the reaction of the halide with hydrogen peroxide is hypochlorous acid (HClO). HClO is most commonly known as household bleach. HClO reacts with a wide variety of biomolecules including deoxyribonucleic acids (DNA), ribonucleic acids (RNA), fatty acid groups, cholesterol, and proteins.
6. Nitric oxide is typically released by vascular endothelium during cerebral ischemia thereby increasing cerebral blood flow. In contrast, neuronal nitric oxide produced cerebral ischemia, when combined with free radicals, results in the production of peroxynitrite which is harmful to neurons.
7. Necrotic neuronal cell death from ischemia results from decreased ATP production by oxidative phosphorylation in the mitochondria. The resulting inability for the neuron to run its membrane ion pumps results in an increase in intracellular calcium and the release of the excitatory neurotransmitter glutamate. Increased intracellular calcium results in the activation of proteases, phospholipases, free radical production, and mitochondrial damage. The resulting disintegration of the cell activates an immune response with the recruitment of macrophages. Macrophages cause increased free radical production, inflammation, and damage to adjacent neurons.
D. Apoptotic cell death
1. Another type of ischemic cell death, apoptosis, results in cell death without the breaking apart of the cell membrane [20]. Apoptosis is a normal phenomenon. There are between 50 and 70 billion cells that die each day due to apoptosis in an average human adult. When the neurons go through apoptosis, there is a lack of an inflammatory response and in contrast to necrotic cell death, no resulting death of adjacent neurons occurs.
2. Apoptosis, the process of programmed cell death, histologically manifests as blebbing, cell shrinkage, nuclear fragmentation, chromatin condensation, and chromosomal DNA fragmentations.
3. Apoptosis produces cell fragments called apoptotic bodies that phagocytic cells can engulf before the contents of the dying cell can leak out and cause inflammation.
4. Apoptosis is controlled by a diverse set of cell signals that have both intracellular and extracellular origins.
5. Dying cells that undergo the final stages of apoptosis display phagocytotic molecules. These molecules mark the cell for phagocytosis by cells possessing the appropriate receptors, such as macrophages.
6. Neuronal ischemia can affect the expression of genes that result in the production of apoptotic or antiapoptotic proteins. Antiapoptotic proteins which help prevent apoptosis include neuronal apoptosis inhibitory protein, heat shock proteins, and Bcl-2 type proteins. Trophic factors can inhibit apoptosis while interleukin 1B and tumor necrosis factor can promote it.
7. Some types of mice with abnormal extrinsic signaling proteins (FasL) show a 93% reduction in infarct volume after experimental middle cerebral artery (MCA) occlusion suggesting a significant contribution of these receptors to cell death in cerebral ischemia [21].
4
E. Autophagy
1. Autophagy is a cellular process required for the recycling of proteins and damaged organelles. Typically, the part of the cell that is to be “autophaged” is surrounded by a membrane separating its contents from the rest of the cytoplasm. This resulting vesicle then attaches to the lysosome, and its contents are digested by lysosomal proteases. In contrast to apoptosis (Type I cell death) and necrotic cell death (Type III cell death), autophagy is described as Type II cell death.
2. Cerebral ischemia is associated with enhanced expression of the autophagy regulator Beclin 1 and subcellular redistribution of the autophagic marker LC3 in ischemic neurons.
3. Prolonged overaction of autophagy can lead to self-destruction of the cell [22].
F. Neurogenesis
1. Evidence suggests that new neurons are generated not only in the neonatal brain but also in the adult brain. The dentate gyrus and the subventricular zone are two areas in the adult brain in which neurogenesis has been identified. The newborn neurons are integrated into neuronal networks and make appropriate connections with target neurons. Exercise, trophic factors (brain-derived neurotrophic factor, epidermal growth factor, and fibroblast growth factor), and neurotransmitters affect proliferation of these neurons. Migrating neuroblasts from the subventricular zone are directed to areas of neuronal ischemic damage.
2. With both focal and global ischemia, neurogenesis significantly increases with peak proliferation between 7 and 10 days postinjury. The majority of the new neurons die. Therefore, their contribution to functional improvement after a stroke remains to be examined.
3. Transplantation of stem cells or their derivatives in animal models of cerebral ischemia can improve function by replacing the lost neurons and glial cells and by mediating remyelination and modulation of inflammation. Transplant cell therapy may work by providing trophic support to the injured tissue and brain fostering both neurogenesis and angiogenesis [23].
G. In summary, ischemia results in a rapid uncontrolled increase in intracellular calcium, resulting in excitatory neurotransmitter release, the activation of lipases, proteases, endonucleases, free radical formation, fatty acid production, nitric oxide production, inflammation, Beclin 1, and the production caspases. All of these can contribute to neuronal cell death [24].
III. Cerebral protection by anesthetic agents
5
A. Mechanism of action
6
1. Anesthetics affect the pathophysiology of cerebral ischemia in many different ways. Anesthetics generally decrease the cerebral metabolic rate by as much as 60% by decreasing neuronal functional activity on the EEG, and can increase the supply of oxygen by cerebral vasodilation. In addition, volatile anesthetics reduce ischemia-induced glutamate release, increase GABA-A–mediated hyperpolarization, antagonize postsynaptic glutamate receptors, and increase levels of antiapoptotic proteins [25,26]. Exposure to the brain by anesthetics, either immediately or up to a few days prior to the induction of ischemia, decreases ischemic brain injury. This is referred to as “preconditioning.” Possible mechanisms for cerebral protection from preconditioning include activation of potassium (K+) channels, production of nitric oxide, activation of adenosine receptors, and activating prosurvival signaling cascades such as serine/threonine protein kinase B (PKB) now called AKT, protein kinase C (PKC), and p38 pathways [27,28].
7
CLINICAL PEARL
Data on ischemic brain neuroprotection from anesthetics has been derived from animal stroke models make it more difficult to form conclusions about the role of inhalational anesthetics in human perioperative cerebral ischemia.
8
2. In spite of extensive work on the basic science of stroke injury mechanisms and countless hours spent on preclinical trial animal stroke models, we have yet to have a single chemical neuroprotection agent. A visit to the stroke trials center (www.strokecenter.org/trials) will show a summary of the clinical trials for stroke. There are no conclusive clinical successes from this list.
B. Barbiturates
1. The ability of barbiturates to provide cerebral protection in focal ischemia has been described in animal studies and one human clinical trial of postcardiopulmonary bypass patients utilizing very high barbiturate doses [29,30]. Another clinical trial did not show that the use of barbiturates in open heart surgery resulted in cerebral protection [31]. Studies have shown that barbiturate doses that are not sufficient to result in an isoelectric EEG are protective in focal ischemia [32].
2. Any combination of decreased cerebral metabolic requirements of oxygen (CMRO2), reverse steal, free radical scavenging, redistribution of cerebral blood flow, blockage of sodium (Na+), potassium (K+), and calcium (Ca++) ion fluxes, scavenging free radicals, decreased intracranial pressure (ICP), and increased regional blood flow are suggested as possible mechanisms for cerebral protection by barbiturates. Studies of the use of barbiturates after cardiac arrest resulting in global ischemia have demonstrated no improvement in clinical outcomes [33]. Another variable to consider is the common drop in body temperature concurrent with the use of the barbiturate.
3. Therefore, barbiturates seem to be protective in models of focal ischemia and in one human clinical trial on cardiac patients.
C. Volatile anesthetics
1. Volatile anesthetics have been shown to be neuroprotective in animal models for focal, hemispheric, and near-complete cerebral ischemia [34,35]. Proposed mechanisms include activation of ATP-dependent potassium channels, upregulation of nitric oxide synthase, reduction of excitotoxic stressors, augmentation of peri-ischemic cerebral blood flow, and upregulation of antiapoptotic factors [36,37].
2. Rat organotypic hippocampus slices treated with isoflurane had substantially less damage when compared with controls [38–40]. In animal models, in rats subjected to hemispheric ischemia, comparing 2% sevoflurane with fentanyl–nitrous oxide (N2O), the sevoflurane group had significantly less damage even out at 28 days [41]. In models in which ischemic brain was evaluated at 2 days postevent, a significant decrease in neuronal death was noted, however, if the ischemic brain was evaluated at 14 days, a reduction in cerebral injury was not observed [42]. This may be due to a lack of protection by volatile anesthetics against ongoing apoptosis. If caspase inhibitors are used along with isoflurane in a focal ischemic model of neuronal injury, sustained neuroprotection is noted [43,44]. The contradiction in the degree of cerebral protection described in these animal models may be secondary to the degree cerebral ischemia used in the model. It may be that cerebral protection in mature adult brains from volatile anesthetics is seen only with mild to moderate ischemia. However, with severe ischemia, cerebral protection by volatile anesthetics may not be possible.
3. Sevoflurane preconditioning for 45 minutes and 60 minutes prior to 1 hour of focal ischemia in a rat model showed a significant decrease in infarct volume, functional outcome, and evidence of apoptosis in the preconditioned animals [45].
4. Recent animal findings suggest that commonly used general anesthetics are damaging to developing neurons and cause significant neuronal deletion in vulnerable brain regions [46–48]. In addition, emerging animal and human data suggest an association between early exposure to general anesthesia and long-term impairment of cognitive development [49].
5. This information, in addition to the fact that most current experimental data on ischemic brain neuroprotection from volatile anesthetics has been derived from animal stroke models makes it more difficult to form conclusions about the role of inhalational anesthetics in human perioperative cerebral ischemia [35].
D. Nitrous oxide
1. Nitrous oxide has been shown to cause a vacuolar reaction, and prolonged exposure can result in neuronal cell death in animal models [46]. Its effect on vitamin B12 metabolism and its lack of effect on metabolic rate seem detrimental.
2. Nitrous oxide probably has a limited role in neuroprotection. Pasternak et al. [50], using the Induced Hypothermia for Aneurysm Surgery Trial (IHAST) database to review delayed ischemic neurologic deficits and 3-month postoperative neurologic or neuropsychological dysfunction in patients delivered nitrous oxide, found no evidence of adverse effects.
E. Propofol
1. Propofol reduces cerebral blood flow, maintains coupling with cerebral metabolic rate for oxygen, and decreases ICP. In vitro and in vivo studies of propofol have shown a neuroprotective effect of propofol in addition to its positive effects on cerebral physiology [51]. Propofol given prior to onset of ischemia, during ischemia, and 3 hours after ischemia, reduces infarct volumes and decreases apoptotic changes and the expression of apoptotic protein Bcl-2 in postperfusion neurons [52,53].
2. In anesthetized animals, propofol showed a similar infarct size when compared to pentobarbital [54]. High dose propofol during focal cerebral ischemia in an animal model when evaluated at 28 days resulted in decreased neurogenesis in comparison to focal cerebral ischemia without propofol [55]. Electroencephalographic burst suppression with propofol during cardiac valve replacement did not significantly reduce the incidence or severity of neurologic or neuropsychological dysfunction [56].
3. Although there is a lack of good clinical human data, animal data does show that propofol is neuroprotective.
F. Etomidate
1. Etomidate works by potentiating the effect of GABA on GABA-A receptors and like barbiturates and propofol can produce suppression of CMRO2. In one model of temporary forebrain ischemia in comparison to 1.1 MAC of halothane and isoflurane or anesthesia with thiopental, there was no protective effect of etomidate seen in the cortex, reticular nucleus of the thalamus, and the striatum. There was some protection in the hippocampus [57,58]. In another investigation of temporary MCA occlusion, the volume of injury was not reduced in comparison to a 1.2 MAC halothane control group versus the etomidate group. In fact the size of volume of injury with etomidate was larger than the control group [57].
2. Etomidate may bind to nitric oxide resulting in decreased cerebral blood flow [57,59]. In patients who have temporary arterial occlusion, administration of etomidate resulted in greater tissue hypoxia and acidosis than equivalent doses of desflurane [60].
3. There is little evidence that etomidate provides cerebral protection.
G. Ketamine
1. Ketamine is an NMDA receptor antagonist. Glutamate excitotoxicity is in part caused by activation on NMDA receptors therefore it is possible that ketamine could provide neuroprotection.
2. Clinical trials with noncompetitive and competitive NMDA receptor antagonists in patients with stroke have shown that these patients develop adverse effects, particularly psychomimetic effects such as hallucinations and agitation [61].
3. In the normal brain, NMDA receptor antagonism results in increased neuronal proliferation. However, in the ischemic brain, NMDA receptor antagonism results in decreased neurogenesis.
4. In both in vitro and animal models of both focal and global ischemia, ketamine has been found to be neuroprotective [62].
5. In a human trial, Nagels et al. [63] could not demonstrate that S(+)-ketamine resulted in greater neuroprotective effects compared with remifentanil during cardiopulmonary bypass procedures when both were combined with propofol.
6. Although there is a lack of good clinical human data, animal data suggest that ketamine is neuroprotective.
H. Benzodiazepines
1. Midazolam is commonly used in anesthesia practice. It works by potentiating the effect of GABA on GABA-A receptors. In animal studies, midazolam has improved neuronal recovery after anoxic and ischemic injuries. High doses of midazolam reduce cerebral metabolic rate and cerebral blood flow. This effect is reversed by flumazenil, a benzodiazepine antagonist [64].
2. Flumazenil has been shown to increase ICP, cerebral blood flow, and cerebral metabolic rate. These facts plus its pro epileptic properties suggest that flumazenil should, as a general rule, be avoided in neurosurgical patients.
I. Lidocaine
1. Lidocaine is a sodium channel blocker. The neuronal depolarization that occurs during cerebral ischemia results in the large flux of sodium into the cell. Lidocaine’s ability to block the sodium channel decreases depolarization and results in a delay in the drop in ATP during cerebral ischemia [65]. Lidocaine also decreases the cerebral metabolic rate for oxygen and inhibits white cell activation to inflammation. Both of these effects may be neuroprotective.
2. In animal models of focal cerebral ischemia, lidocaine reduced infarct size and has shown improved neurologic outcome following focal cerebral ischemia [66,67]. In addition, administration of lidocaine after a 45-minute delay after ischemia resulted in improved survival of neurons in the ischemic penumbra. Lidocaine administered 30 minutes before ischemia and up to 60 minutes after ischemia improved the survival of pyramidal neurons after temporary global ischemia. This enhanced survival was seen at both 1 week and 4 weeks after the ischemic insult [67].
3. Small clinical studies have found improved cognitive outcomes following cardiac surgery in patients to whom lidocaine was administered during their cases. In a follow-up double-blinded intention to treat trial, 158 cardiac surgery patients received a 12-hour infusion of lidocaine started at induction and referenced to a control group. Lidocaine was not found to result in improved scores on neurocognitive examinations at 10 and 25 weeks postanesthesia [68].
4. Lidocaine is probably neuroprotective. Its clinical utility is under investigation.
IV. Cerebral protection by nonanesthetic agents
A. Calcium channel blockers
1. Voltage-gated calcium channels have a central role in dendritic development, neuronal survival, synaptic plasticity, activation of gene transcription, and are essential to convert electrical activity to biochemical processes in the brain.
2. Neurons express at least nine types of voltage-gated calcium channels specialized for different functions [69]. Some studies of dihydropyridine, phenylalkylamine, and benzothiazepine calcium channel blockers in both in vitro and animal models of cerebral ischemia have shown neuronal protection. However, this data is variable [70,71]. Nimodipine, a dihydropyridine which blocks L-type calcium channels, has been shown to increase recovery after subarachnoid hemorrhage [72]. In contrast, it has been demonstrated that prolonged exposure by cultured neurons to various antagonists of L-type voltage-gated calcium channels can induce apoptosis. In fact, activating L-type voltage-gated calcium channels, in vitro and in animal model studies of neuronal ischemia have actually reduced neuronal injury [71].
3. A large clinical trial of the use of nimodipine after stroke was discontinued due to higher mortality in the nimodipine group [73]. With the exception of nimodipine for subarachnoid hemorrhage, calcium channel blockers do not seem clinically useful in ischemic neuroprotection.
B. Magnesium
1. Magnesium blocks various voltage-gated and transmitter-activated channels. The NMDA receptor is normally blocked by magnesium ions and will only respond to glutamate when this magnesium-induced block is removed on depolarization [74–76]. Magnesium blocks the influx of calcium and other ions.
2. In animal models of focal ischemia, magnesium has been shown to decrease levels of proapoptotic proteins [77].
3. Magnesium crosses the blood–brain barrier poorly and therefore has limited access to the central nervous system in the adult. Animal models have not reproducibly shown a reduction in infarct volume [78,79]. A clinical trial showed no improvement in outcome with the intravenous use of magnesium as therapy after stroke [80]. In addition, a human study of magnesium given before very preterm birth to protect the infant brain did not yield significant improvement with magnesium treatment [81].
4. Magnesium infusion has been shown to improve outcome when used in a postnatal fashion in term neonates with severe perinatal asphyxia [79].
5. At this point, there seems limited efficacy in the use of magnesium after cerebral ischemia except, perhaps, in the neonate.
C. Free radical scavengers
1. Both necrotic and apoptotic cell damage are thought to be secondary to free radical damage secondary to cerebral ischemia. Tirilazad, NXY-059, alpha-tocopherol, and N-tert-alpha-phenyl-butyl nitrone have been shown in animal studies to improve outcome after cerebral ischemia.
2. Tirilazad is a nonglucocorticoid, 21-aminosteroid that inhibits lipid peroxidation. Studies in experimental models of ischemic stroke had suggested that tirilazad had neuroprotective properties.
3. NXY-059 is a free radical trapping agent that is neuroprotective in animal models of stroke.
4. Tirilazad and NXY-059 did not show improved outcome in reproducible clinical trials after cerebral ischemia [82–84].
D. Excitatory amino acid modulators
1. Drugs that modulate glutamate action either by inhibiting its release or by blocking postsynaptic receptors could be neuroprotective agents [85].
2. Gavestinel (GV-150,526) is a drug which acts as an NMDA antagonist, binding selectively to the glycine site on the NMDA receptor complex. It is neuroprotective in animal studies and progressed to Phase II clinical trials in humans before being dropped for lack of efficacy [86].
3. Lubeluzole acts as an indirect NMDA antagonist. It inhibits the release of glutamate, inhibits nitric oxide synthesis, and blocks calcium- and sodium-gated ion channels. At high doses, Lubeluzole increases the Q–T interval therefore limiting its dose. Lubeluzole was not efficacious in clinical trials [85].
E. Trophic factors, erythropoietin, and cytokines
1. Neurons have receptors for trophic factors such as brain-derived growth factor and nerve growth factor. These are required for neuron survival. They phosphorylate amino acids via receptors that inhibit apoptosis. Ischemia can cause a reduction in the production of trophic factors and therefore trigger apoptosis.
2. In addition to its effect on erythropoiesis, erythropoietin may protect neurons from ischemia by acting as a trophic factor, activating antiapoptotic pathways, promoting angiogenesis, and decreasing inflammation. Erythropoietin crosses the blood–brain barrier poorly and therefore its ability to enter the brain may be dose dependent. In animal models for focal cerebral ischemia, systemic administration of erythropoietin has decreased infarct size [87,88]. Clinical trials using erythropoietin in the early postnatal period in very low birth weight infants have been associated with improved long-term neurologic and cognitive outcomes [89,90]. Erythropoietin has been shown to be safe and effective in clinical trials [91]. However, a recent double-blinded controlled randomized German multicenter stroke trial found no favorable effects with the use of erythropoietin. In addition, they found an increased death rate in the erythropoietin-treated group especially when the stroke patients were pretreated with thrombolytics [92,93].
3. Cytokines, such as tumor necrosis factor and interleukin 1B are immune system activators and can affect ischemic neuronal damage [94,95]. More research needs to be done in this area.
9
F. Statins
1. Statins are either synthetic- or fermentation-derived HMG-CoA reductase inhibitors. There is evidence that statins have beneficial effects within the cerebral circulation and the brain parenchyma during ischemic stroke and reperfusion. Statins upregulate endothelial nitric oxide synthase, attenuate inflammatory cytokine nitric oxide synthase, and possess antioxidant properties.
2. Human trials have shown a 16% reduction in strokes if treated with statins within the prior 1 to 6 months in patients with no known cardiac disease [96]. Studies have demonstrated that unlike those with prior ischemic stroke or transient ischemic attack (TIA), patients with prior hemorrhagic stroke had no statin-associated reductions in either recurrent stroke or major cardiovascular events. In fact, an increased risk of hemorrhagic stroke, particularly in patients treated with thrombolytics has been described [97,98].
3. Therefore, the use of statins must be used with caution after hemorrhagic stroke.
CLINICAL PEARL
Human trials have shown a reduction in strokes if treated with statins within the prior 1 to 6 months in patients with no known cardiac disease.
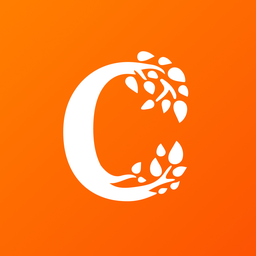
Full access? Get Clinical Tree
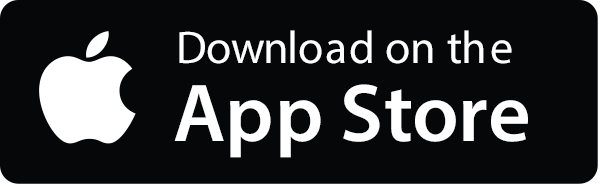
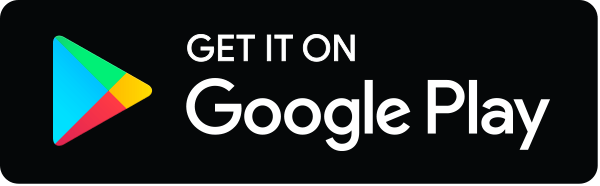