Introduction
Stereotactic surgery is a minimally invasive surgical intervention used for the treatment of functional disorders and in the diagnosis of intracranial lesions. The anesthesiologist plays a key role in the management of patients undergoing stereotactic surgery. The techniques of anesthesia will vary depending on the patient and the traditions and requirements of each institution performing these procedures. The purpose of this chapter is to familiarize the anesthesiologist with the surgical techniques for the insertion of deep brain stimulation (DBS), the influences of anesthesia agents on this procedure, and the anesthetic management of these patients. New developments in this field, as well as in the management of patients for other stereotactic procedures such as brain biopsy and radiosurgery, will also be discussed.
Stereotactic surgery
Stereotactic surgery requires a planning system with multimodality neurological imaging and calculation of coordinates of an intracranial location or lesion. This can be performed with either a frame-based or a frameless system. A stereotactic headframe system such as Cosman-Roberts-Wells or Leksell uses a three-dimensional coordinate’s frame and a multipurpose stereotactic arc that locates small targets inside the brain. Computerized tomography (CT) or magnetic resonance imaging (MRI) with or without angiography is used to identify the targeted lesion in relation to the distance from the lesion to reference points on the external frame. Surgical apparatus such as an electrode guide or biopsy needle is attached to the headframe and adjusted according to the three-dimensional coordinates of the target. Frameless stereotactic surgery avoids the invasive placement of the headframe and uses external fiducial scalp markers for reference. The relationship of the surgical instrument is then displayed over a previously obtained image of the brain. After imaging and the calculation of coordinates, the patient is transferred to the operating room for surgery.
Stereotactic surgery
Stereotactic surgery requires a planning system with multimodality neurological imaging and calculation of coordinates of an intracranial location or lesion. This can be performed with either a frame-based or a frameless system. A stereotactic headframe system such as Cosman-Roberts-Wells or Leksell uses a three-dimensional coordinate’s frame and a multipurpose stereotactic arc that locates small targets inside the brain. Computerized tomography (CT) or magnetic resonance imaging (MRI) with or without angiography is used to identify the targeted lesion in relation to the distance from the lesion to reference points on the external frame. Surgical apparatus such as an electrode guide or biopsy needle is attached to the headframe and adjusted according to the three-dimensional coordinates of the target. Frameless stereotactic surgery avoids the invasive placement of the headframe and uses external fiducial scalp markers for reference. The relationship of the surgical instrument is then displayed over a previously obtained image of the brain. After imaging and the calculation of coordinates, the patient is transferred to the operating room for surgery.
Deep brain stimulation
Introduction
Deep brain stimulation is used for the treatment of patients with neurological disorders that have an alteration of function that is not usually accompanied by gross structural or anatomical changes, such as Parkinson’s disease. The aim of this procedure is to improve the quality of life of the patient. The overall procedure for the insertion of a DBS includes the placement of electrodes into deep brain structures for microelectrode recordings (MER) and macrostimulation for clinical testing of the patient and then the connection of the DBS to an implanted pulse generator.
History
Historically, surgical procedures for functional neurological disorders, such as Parkinson’s disease, consisted of making a lesion in deep brain structures with procedures such as thalamotomy, pallidotomy and cingulotomy. , These procedures are irreversible and may be associated with permanent side effects. In 1987 the use of DBS was first described as an alternative treatment to ablative procedures to reduce the symptoms of patients with Parkinson’s disease. The DBS system has advantages over surgical ablation because it is nondestructive, reversible, and adjustable. The safety and long-term benefits of DBS are well documented. The DBS used today provides multiple programmable options, which allow for long-term control of symptoms.
Indications
Following the initial success of DBS therapy in patients with Parkinson’s disease, the indications and applications have expanded to include many other disorders. Movement disorders treated with DBS include Parkinson’s disease, dystonia, essential tremor, and Tourette’s syndrome, and psychiatric disorders include depression, obsessive compulsive disorder, and anorexia. Other indications are chronic pain, epilepsy, Alzheimer’s disease, and dementia. With future advances in research in DBS, the list of indications will continue to be modified and extended.
Mechanism and Targets
The exact mechanism of the DBS is not fully understood and may well differ depending on the site of stimulation. The primary target sites vary with the patient’s symptoms and include the subthalamic nuclei (STN), globus pallidus pars interna (GPi), and the ventralis intermedius nucleus of the thalamus (Vim). The effects of stimulation on the various nuclei differ. Stimulation of the STN causes hyperpolarization or “neuronal jamming” and this action results in the inhibition of its activity. Stimulation of the GPi nuclei may result in activation of gamma amino butyric acid (GABA)ergic axons, which in turn inhibits GPi neurons. In contrast, stimulation of the Vim nucleus of the thalamus activates output to neurons in the reticular nucleus, which results in an inhibitory signal being sent to thalamic nuclei. The inhibition of these targeted nuclei will then result in improvements of the patient’s symptoms ( Fig. 18.1 ).
The most common target for DBS in Parkinson’s disease is the STN, as this ameliorates the cardinal symptoms like bradykinesia, rigidity, and tremor. The effective target for stimulation in patients with generalized dystonia and cervical dystonia is the GPi nuclei. Stimulation of the GPi is also used in patients with Parkinson’s disease with severe dyskinesia. Stimulation of the Vim nuclei is effective for essential tremors and tremor- dominant Parkinson’s disease. Subcallosal cingulate gyrus (Cg25), anterior limb of internal capsule (AIC), and the nucleus accumbens (NAcc) are the common target areas in patients with depression and obsessive compulsive disorders. Other targets for stimulation include anterior thalamus (epilepsy), centromedian–parafascicular thalamic nuclei (Tourette syndrome), fornix, and hypothalamus (dementia).
Deep Brain Stimulator Device
The DBS system consists of three components: the intracranial electrode, an extension cable and an implanted pulse generator. The electrode is a coiled wire insulated in polyurethane with four platinum iridium electrodes for implantation in the target neural tissue. The lead is connected by an extension cable to the implanted pulse generator which is a battery-powered programmable single- or dual-channel internal pulse generator (IPG) with a battery unit encased in a titanium housing that sends electrical pulses to stimulate the target site. It is placed subcutaneously below the clavicle or in the abdomen and can be programmed to optimize symptom suppression and control side effects.
Surgical Techniques
The therapeutic effectiveness of DBS depends on the placement of the stimulating electrodes in close proximity to the target nuclei. The target nuclei are deep seated and small in size, requiring innovative methods to increase the accuracy of placement. This includes the use of imaging techniques to visualize and establish coordinates to the target nuclei, intraoperative electrophysiological guidance using MER, and macrostimulation testing of an awake patient to verify that the stimulation of the electrode improves the symptoms with minimal side effects. The procedure begins with placement of the headframe to the patient’s skull followed by imaging. Usually MRI is performed to identify target nuclei, although, alternatively, CT can be used if MRI is not possible or is contraindicated. The patient is transferred to the operating room and positioned in a sitting or semi-sitting position with the headframe fixed to the operating room table. The incision and burr hole(s) are made to allow for the placement of an electrode guide and then the DBS electrode(s) into the vicinity of the planned target. Many centers utilize MER to further fine-tune localization of the target sites by detecting and amplifying the activity of individual neurons. The MER electrode is usually inserted 10–15 mm above the target site and then advanced in steps of 0.5–1 mm with a micro driver along the trajectory toward the target nuclei while spontaneous neuronal discharges are recorded. Specific brain structures can be identified based on their unique patterns of firing. Using these variations in spontaneous firing rates between specific nuclei, as well as changes in the firing rates related to patient movement, the neurophysiology team is able to localize the specific brain target ( Fig. 18.2 ). This allows feedback along the entire trajectory and fine adjustments of position before inserting the final electrode. Macrostimulation, which involves the clinical testing of the patient’s movements, is used to verify that the stimulation of the electrode at this location will improve their symptoms and not cause any side effects. Radiological confirmation of the electrode placement is obtained and then it is secured and the wound closed. Imaging techniques using 3 Tesla MRI will also improve the accuracy of target localization. Successful insertion of a MRI guided STN-DBS has been used in patients with Parkinson’s disease.
Internalization of the Electrodes and Implantation of Pulse Generator
The second stage, the internalization, is performed by tunneling the electrode(s) and connecting extension cable through the scalp and then subcutaneously tunneling down the side of the neck to where it is connected to the internal pulse generator and battery unit, which is usually implanted in the infraclavicular region of the chest. This procedure may be performed on the same day as insertion of the DBS or maybe delayed for 3 days up to 2 weeks post initial procedure. There is no evidence to favor the best timing of the second stage. The timing depends on the duration of the initial procedure and on patient cooperation. Another reason for delaying is the development of a “microlesion” effect due to edema around the freshly implanted electrode. This effect may cause improvement of the patient’s symptoms without any stimulation, which impairs the ability to check for stimulation-induced benefits.
The Effects of Anesthetic Agents on Neuromonitoring for Target Localization
Microelectrode Recordings
The MER are the direct measurements of both spontaneous and stimulus-evoked cellular activity. They help to distinguish the areas that are populated by neurons from the axonal tracts, which are devoid of any action potential activity. The MER are obtained as the microelectrode is passed along the trajectories to the target nuclei. Precise localization can be achieved with a single trajectory pass but multiple passes may be needed. Accurate localization of the target nuclei depends on the individual cellular activity as well as the background neuronal discharges. There are differences in the rate and the pattern of neuronal discharges among the various target cells. Anesthetic agents have been shown to affect both the background activity and the neuronal spike activity.
The effect of anesthetic agents on the MER varies with both target nuclei and also within the same nuclei with different disease processes, such as Parkinson’s disease and dystonia. The ability to obtain MER from the STN nuclei during anesthesia has been more successful. The anesthetic techniques used have varied from conscious sedation with propofol and dexmedetomidine to general anesthesia with either intravenous or inhalation techniques. Propofol has been shown to affect the background neuronal discharges and both propofol and remifentanil have been shown to produce only minor alterations of STN discharge activity. , Similarly, dexmedetomidine has been shown to have minimal effect on the MER from the STN. The degree of MER suppression is related to the level of sedation.
The MER from the GPi is often difficult to obtain under general anesthesia. Anesthetic agents have minimal effect on MER from the GPi in patients with Parkinson’s disease. , By contrast, the firing rates in the GPi are substantially decreased in patients with dystonia under general anesthesia with propofol compared to patients with no sedation. The differences in the anesthetic effects on the various target nuclei (STN versus GPi) may be explained by the amount of their GABA input. Neurons from the GPi predominantly receive gamma-amino butyric acid (GABA) input from putamen and GPe and hence they are more suppressed by propofol. Successful target localization in patients with dystonia is possible using balanced anesthesia with low-dose propofol in combination with low level of sevoflurane and/or desflurane. ,
Macrostimulation Testing
Macrostimulation testing of the patient is another complimentary technique for target localization, but requires an awake and cooperative patient. By stimulating the target areas, the clinical benefit of DBS can be assessed by observing the improvement in clinical symptoms such as tremors and rigidity. With stimulation of adjacent structures (internal capsule, medial lemniscus), the patient can report subjective symptoms such as paresthesia or abnormal motor activity. The electrode position can be adjusted to minimize any side effects. When sedation is provided, short-acting agents should be used and all sedation stopped well before the testing. General anesthesia interferes with the evaluation of clinical benefits of DBS by the suppression of clinical symptoms such as tremors and rigidity. , Also, the patient cannot report subjective effects such as paresthesia or abnormal motor activity associated with stimulation of adjacent structures. Some centers that routinely perform DBS insertion under general anesthesia do not conduct any form of stimulation testing in the operating room but confirm the location of implanted DBS electrode with MRI. Currently, there are no data to show the differences in adverse effects with this approach.
Anesthesia for Deep Brain Stimulation
Goals
The goals of anesthesia during insertion of DBS include providing optimal surgical conditions and patient comfort during the procedure, facilitating intraoperative monitoring and target localization, and rapid diagnoses and treatment of any complications. Considerations for the choice of anesthetic agents and techniques used for each patient include the specific patient population and the specific DBS target. , , , The anesthetic techniques vary according to the institutional practice and have included monitored anesthesia care or conscious sedation with local anesthesia, and general anesthesia. Currently, none of the anesthetic techniques has proven to be superior to others, although awake or sedation techniques are popular as they facilitate intraoperative neurophysiological monitoring. ,
Preoperative Evaluation and Preparation
Successful treatment with DBS is dependent upon appropriate patient selection and evaluation by a multidisciplinary team of neurologists, neurosurgeons, neurophysiologists, and psychiatrists. This includes assessment of the patient with respect to diagnosis, cognitive and psychiatric status, access to care, and expectations by the patient, and the patient’s response to medical treatment. , All patients should also be seen by the anesthesiology team prior to surgery. This includes the routine preoperative assessment and preparation of any patient for a neurosurgical procedure. The considerations for the anesthetic management of patients are shown in Box 18.1 . Many patients may have comorbidities related to the disease processes for which the DBS is indicated ( Box 18.2 ). , Patients who will be awake need good psychological preparation and information on what to expect during the procedure. Instructions for the continuation of disease-specific drugs should be done in conjunction with the neurosurgical team, as some patients need to be in a “drug-off” state to facilitate MER and clinical testing. If the patient’s symptoms, especially in Parkinson’s disease, worsen during the “drug-off” state a reduced dose of their regular medication can be administered.
- 1.
Preoperative preparation and reassurance of patient
- a.
Appropriate patient selection for anesthesia technique (awake, general anesthesia)
- b.
Long procedure – positioning, neurological testing
- a.
- 2.
Patient-related concerns
- a.
Primary disease (Parkinson’s, dystonia)
- b.
Comorbidities (cardiac, respiratory, diabetes)
- c.
Age (elderly, children)
- a.
- 3.
Medications
- a.
Polypharmacy, altered pharmacokinetics and dynamics
- b.
Medication “off state” – worsening of symptoms
- c.
Continuation of medications
- a.
- 4.
Procedure and intraoperative considerations
- a.
Multiple locations for procedure and transporting between (radiology, operating room)
- a.
- 5.
Stereotactic frame
- a.
Airway considerations
- a.
- 6.
Positioning
- a.
Sitting, semi-sitting
- b.
Complications (venous air embolism, hypovolemia)
- a.
- 7.
Blood pressure control
- a.
Treatment of hypertension
- a.
- 8.
Anesthetic effects on microelectrode recordings
- 9.
Stimulation testing of patient
- a.
Need cooperative, alert patient
- a.
- 10.
Postoperative considerations
- a.
Slow emergence
- b.
Postoperative cognitive problems
- a.
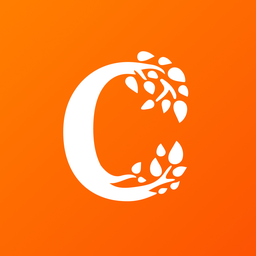
Full access? Get Clinical Tree
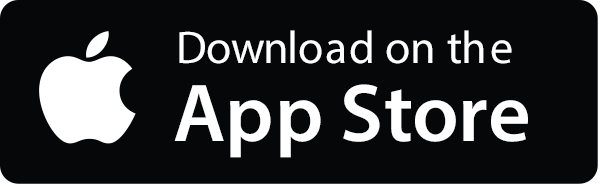
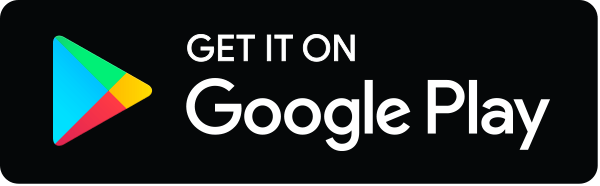
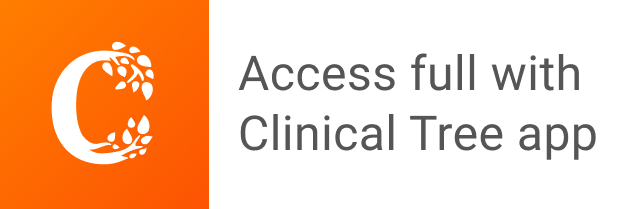