In general, each vertebra consists of a cylindrically shaped body, a vertebral (or neural) arch, the (dorsal) spinous process and transverse processes at each side (Fig. 4.2). The spinous and transverse processes serve as attachment points for muscles and ligaments. Such muscles and ligaments account for stability and movements, especially of the head and neck. There are three spinal ligaments that run along the entire length of the spine: the anterior and posterior longitudinal ligaments which line the anterior and posterior aspects of the vertebral bodies, and the supraspinous ligament which joins the tips of the spinous processes (Fig. 4.3). Spinal ligaments are in general pre-stressed in neutral position. As for the muscles, superficial, intermediate and deep muscles are distinguished. They are symmetrical about the sagittal plane, i.e. all muscles appear in pairs. The deep muscles are closely attached to the vertebrae, while the intermediate muscles account for longer distances connecting, for example, the neck to the thorax and the skull. The superficial muscles, on the other hand, have no direct attachments to the spinal column.
The foramina vertebralia of all vertebrae form the spinal canal that includes the spinal cord and the associated soft tissues (Fig. 4.4). The spinal cord itself is surrounded by cerebrospinal fluid (CSF). Blood vessels, especially venous vessels, are also present within the spinal canal.
In the cervical spine, it has to be noted that vertebrae C1 and C2 are anatomically different from other vertebrae. C1, also called atlas, comprises a bony ring with large articulated surfaces only. Together with the second cervical vertebra, which is characterized by a dominant process (dens axis) on its upper side, they form the atlanto-axial joint. Consequently, there is no intervertebral disc between C1 and C2 (Fig. 4.5).
With respect to physiological neck motion, four basic movements are possible: flexion, extension, lateral bending and (axial) rotation (Fig. 4.6) and combinations thereof. To allow this motion, different joints can be identified. Apart from the atlanto-axial joint which is responsible for head rotation, the intervertebral joints, in particular the intervertebral discs, by nature of their fibre-enforced annulus and the viscous nucleus, transmit (compression and shear) forces and moments. Furthermore, the motion is guided by the two sets of facet joints (also called zygapophyseal joints) of each vertebra.
4.2 Injury Mechanisms
Assessing the threat to life, the AIS (Abbreviated Injury Scale) grades several spinal injuries (Table 4.1). Injuries to the upper cervical spine are generally considered to be more serious and life threatening than those at a lower level (Viano 2001).
AIS code | Description |
---|---|
1 | Skin, muscle: abrasion, contusion (hematoma), minor laceration |
2 | Vertebral artery: minor laceration |
Cervical/thoracic spine: dislocation without fracture | |
Thoracic/lumbar spine: disc herniation | |
3 | Vertebral artery: major laceration |
Cervical/thoracic spine: multiple nerve root laceration | |
4 | Cervical/thoracic spine: spinal cord contusion incomplete |
5 | Cervical/thoracic spine: spinal cord laceration without fracture |
6 | Decapitation |
Cervical spine: spinal cord laceration at C3 or higher with fracture |
In principle, injuries to the cervical spine can be classified according to the possible motion of the neck and possible mechanical loading (Figs. 4.6 and 4.7). Shear in antero-posterior direction and axial torsion may cause dislocation of the atlanto-occipital joint, while large compression might result in fracture of the atlas (C1) thereby breaking C1 into two to four sections (Jefferson’s fracture, Fig. 4.8). Isolated atlas fractures are common after axial loading; however, 40–44 % of atlas fractures have concomitant axis fractures (Kakarla et al. 2010). If axial compression is combined with extension of the neck, C2 fractures, commonly known as hangman’s fractures, can occur. In a vehicle accident this type of fracture is often related to an unrestrained occupant whose forehead or face impacts, for example, the windscreen.
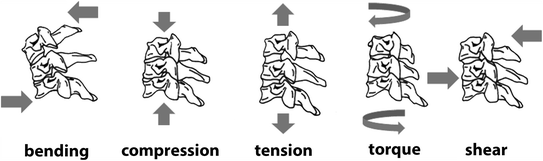
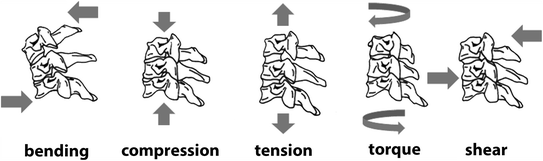
Fig. 4.7
Possible loading of the neck includes compression of the neck, tension (force stretching the neck), shear (force perpendicular to the neck column), flexion moment (forward bending of the neck), extension moment (rearward bending of the neck) and axial torsion (adapted from McElhaney et al. 2002)
In automotive crashes, loading of the neck is generally due to head contact forces and combined axial or shear load with bending. Because of the anatomical curvature of the cervical spine, bending in whichever direction is almost always present. While pure compression may result in fractures as described above, non-contact head acceleration and airbag deployment are suspected to be the cause of pure tension injuries of the upper cervical spine (e.g. McElhaney et al. 2002). Lesions resulting from tensile loading include dislocation of the occipital condyles, ligamentous injury and fractures, e.g. dens fracture (e.g. Nightingale et al. 1998).
However, as head contact is frequently observed, the following neck injury modes are considered predominant: compression-flexion, compression-extension, tension-flexion, tension-extension and lateral bending.
Wedge fractures of the anterior vertebral bodies result from a combination of flexion bending moment and axial compression force of the neck. Head rotation often accompanies this loading scenario, but is not essential. With increasing load, burst fractures and fracture dislocation of the facets can occur (Fig. 4.9). The latter two conditions are unstable and potentially disrupt or injure the spinal cord. Hereby the extent of the injury depends on the penetration of the vertebral body or its fragments into the spinal canal.
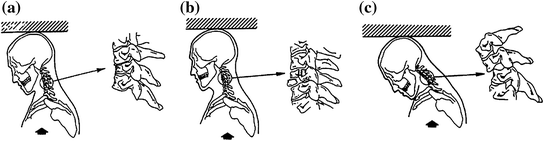
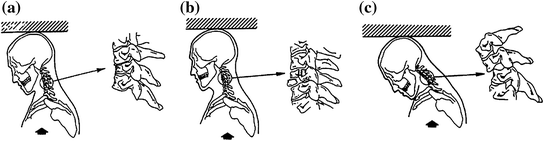
Fig. 4.9
Compression-flexion injury mechanism can result in wedge fractures (a), burst fractures (b), or bilateral facet dislocation (c). Although the figure illustrates head rotation as part of the injury mechanism, research has shown that head rotation needs not accompany this injury (adapted from McElhaney et al. 2002)
Compression-extension loading produces fractures of the posterior structures of the neck in both the upper and lower regions (e.g. Pintar et al. 1995; Nightingale et al. 1997). As indicated in Fig. 4.10, frontal impact to the head with the neck in extension is likely to cause compression-extension loading.
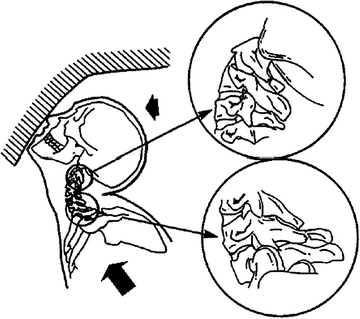
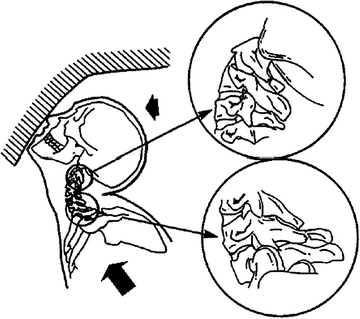
Fig. 4.10
Compression-extension mechanism: compression of the cervical spine can be enforced by inertia forces due to the body being moving towards the head (adapted from Goldsmith and Ommaya 1984)
Frontal impact in which the torso is restrained and the neck is meant to stop head movement can result in flexion of the cervical spine while being subjected to tension. Bilateral facet dislocation was observed after such loading. However, it should be noted that such injury may also result from compression-flexion loading, suggesting that the magnitude of the bending moment rather than the axial load seems to be the determining factor.
Tension-extension loading is the underlying mechanism for several injuries (Fig. 4.11). It commonly occurs when unbelted occupants hit the windscreen or when the chin impacts the dashboard. In both cases the head rotates rearward and tensile force and an extension moment are applied on the neck. In case of hitting the windscreen with the head, also a hangman’s fracture of C2 can occur. Furthermore, soft tissue neck injuries are hypothesised to result from tension-extension loading. A more detailed discussion of this type of injury is presented further down in this section.
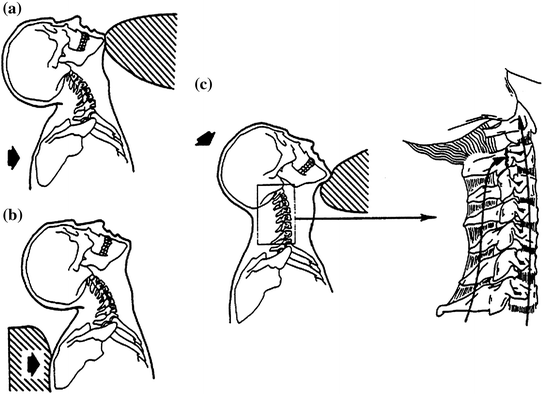
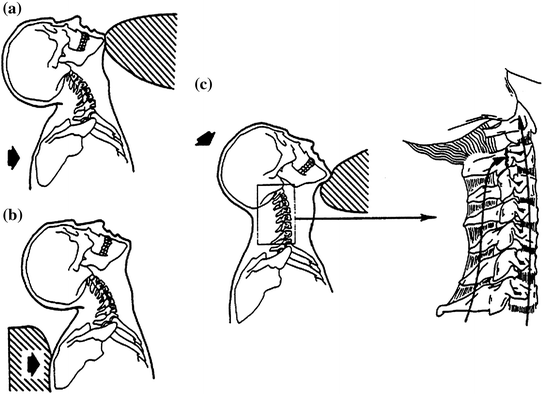
Fig. 4.11
Tension-extension loading primarily occurs through (a) fixation of the head with continued forward displacement of the body, b inertial loading of the neck following an abrupt forward acceleration of the torso, and c forceful loading below the chin directed postero-superiorly (adapted from McElhaney et al. 2002)
Injuries due to lateral bending are, for instance, observed after automotive side impact. Axial loads (e.g. compression) or shear loads are often associated with lateral bending (Fig. 4.12). Lateral wedge fractures of the vertebral body and fractures to the posterior structures on one side of the vertebral column can result. Additionally, lateral bending in combination with torsional loads may occur. Such cases possibly lead to unilateral facet dislocations or unilateral locked facets (Moffat et al. 1978). However, pure torsional loads on the neck are rarely encountered in automotive accidents (Viano 2001).
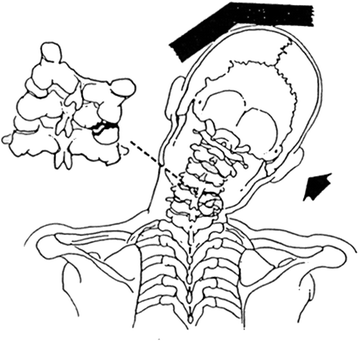
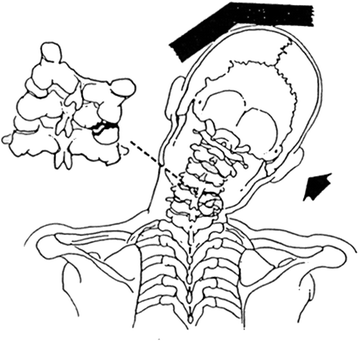
Fig. 4.12
Lateral bending and compression resulting in fracture on the compressed side (adapted from Vetter 2000)
Soft tissue neck injuries are by far the most frequent injuries of the spine that are sustained in automotive accidents. Epidemiological data shows that females have a higher risk of sustaining such injuries than males (e.g. Viano 2003; Linder et al. 2008, 2012). Soft tissue neck injuries are reported from low-speed rear-end collisions, as well as from frontal and frontal-oblique collisions involving belted occupants, with and without head contact. The symptoms are diverse ranging from neck pain, headache, numbness, dizziness to visual disorders and neurological deficiencies (e.g. Ferrari 1999). In many cases no lesions are evident even if advanced diagnostic measures are applied, and therefore such injuries are most often classified as minor (AIS1). Such a classification using the AIS scale is rather broad. To allow for a more detailed assessment of soft tissue neck injuries, the Quebec Task Force (Spitzer et al. 1995) established another injury scale, categorising the symptoms and signs into four grades according to the clinical presentation (Table 4.2).
Table 4.2
Classification of WAD as proposed by the Quebec Task Force (adapted from Spitzer et al. 1995)
Grade | Clinical presentation |
---|---|
0 | No complaint about the neck |
No physical sign(s) | |
1 | Neck complaint of pain, stiffness or tenderness only |
No physical sign(s) | |
2 | Neck complaint and musculoskeletal sign(s) |
3 | Neck complaint and neurologic sign(s) |
4 | Neck complaint and fracture or dislocation |
Regarding the injury mechanism, hypotheses have to rely on experiments (see Sect. 4.3) or on symptomatic clinical observations. Due to the complex anatomy of the cervical spine various vulnerable structures are assembled in a quite small area. Hence, different tissues were proposed to be the cause for neck pain. Many studies have suggested that ligaments and muscles are injured. The zygapophyseal joint is also often suspected to cause complaints. Also nerve tissue injuries, in particular in the vicinity of the spinal ganglia, are reported. Further hypotheses can be found identifying other tissues (e.g. vertebral arteries, intervertebral discs), but these suggestions are discussed very controversially (cf. e.g. Ferrari 1999; Curatolo et al. 2011).
Analysis of the motion of the neck during rear-end or frontal collisions (both may to result in soft tissue neck injuries) reveals complex kinematic sequences including various mechanical loading conditions. The resulting movement of a car occupant during a rear-end collision (i.e. the vehicle is struck from behind and thus is accelerated forward), can for example be divided into three different phases (Fig. 4.13). In the retraction phase, an occupant who is sitting upright in the driver’s position is pushed forward by the seat back. The contact and force transmission occurs primarily in the shoulder area. Due to its inertia, the head, which is not in contact with any structure of the car, has a tendency to keep its state of motion. Relative to the occupant, the head lags behind the torso. It moves backwards without any rotation about the lateral axis, i.e. it retracts. Hence, the upper cervical spine is forced into flexion mode and the lower cervical spine into extension. This deformation of the neck, called S-shape formation, is regarded as crucial for the injury mechanism. The presence of the S-shape is well established today and was observed in various experiments using dummies equipped with specially designed necks as well as volunteers and cadavers (cf. e.g. Ono and Kaneoka 1997; Ono et al. 1998; Eichberger et al. 1998; Grauer et al. 1997; Svensson et al. 1993; Wheeler et al. 1998; Yoganandan and Pintar 2000; Ivancic and Xiao 2011). Following the S-shape formation, the head starts rotating backwards which in turn leads to the extension of the entire cervical spine. Eventually, the retraction phase is concluded by maximum extension which depends on impact severity, the presence of a head restraint and the individual physiological limitations of the occupant.
The next phase is a forward movement which is characterised by a change in the direction of the movement, i.e. head, neck and torso move now forward. This phase is strongly influenced by the seat design, in particular by the elasticity of the seat and the corresponding rebound effect (Muser et al. 2000; Viano et al. 2013). When the occupant returns to a position which, in sagittal direction, equals the initial position prior to the impact, the forward motion phase is completed and the belt restraint phase follows. The belt system restrains the occupant such that the thoracic spine is stopped while the head continues to move forward. Consequently, an inverse S-shape of the neck can be observed. However, due to damping of the restraining forces by the thoracic cage, this effect is less pronounced than the S-shape experienced first. Additionally, since the (ventral) belt contact point is lower than the (dorsal) shoulder contact point, more vertebrae participate in this second S-shape formation, thus allowing for smaller loads on the individual vertebrae. Finally, an overall flexion of the cervical spine concludes the kinematic phases specifically related to rear-end collision.
For frontal collisions (without head contact) similar kinematic phases including the inverse S-shape apply.
Considering the complex occupant kinematics, it is not surprising that different mechanisms are discussed as causes for soft tissue neck injuries (e.g. Walz and Muser 1995). A shearing movement of the vertebrae has, for instance, been related to lesions of the facets of the intervertebral joints (Yang et al. 1997). Hyperextension of the neck, i.e. an exceedance of maximum neck moments and head excursion angles (Mertz and Patrick 1971), is also considered to be a possibility. However, due to head restraints such a mechanism has become rare. Additionally, a hypothesis proposed by Aldman (1986) takes into consideration a pressure gradient that develops in the venous and cerebrospinal fluid of the spinal canal causing cellular injuries (Svensson et al. 1993; Schmitt 2001).
In summary, many causes for soft tissue neck injuries are hypothesised, but the underlying mechanisms are difficult to elucidate and are therefore not fully understood, let alone scientifically proven. In practice, the S-shape deformation is regarded to play a crucial role when discussing possible injury mechanisms. Consequently, injury criteria based on the S-shape deformation have been established (see Sect. 4.4) and new dummies have been developed that are capable of a biofidelic reproduction of the relative motion between the head and the torso (see Sect. 2.6.1).
Injuries to the thoracolumbar spine sustained in automotive crashes are rare and play a minor role compared to cervical spine injuries. However, back pain is commonly reported after collisions and severe injuries to the spinal cord may, of course, also occur. King (2002) identifies seven different types of thoracolumbar spine injuries: anterior wedge fractures of the vertebral bodies, burst fractures of vertebral bodies, dislocations and fracture-dislocations, rotational injuries, Chance fractures, hyperextension injuries and soft tissue injuries. With respect to automotive accidents, anterior wedge fractures result when combined flexion and axial compression loading arise. This may happen, for example, in severe frontal impacts when the shoulder harness imposes a large load across the torso, causing the curved thoracic spine to straighten out. Experiments conducted with cadavers and volunteers that were restrained by a three-point belt showed that a compressive force is generated in the thoracolumbar spine which might cause wedge fractures (Begeman et al. 1973). Principally these injuries occur at all levels of the thoracolumbar spine, but are most likely between T10 and L2 (King 2002). Anterior wedge fractures are also observed in aircraft accidents, particularly when ejection of the pilot is involved. Historically, the analysis of the pilot ejection problem was the primary motivation to study injuries of the thoracolumbar spine.
Chance fractures (named after G. O. Chance who first described this type of fracture in 1948) are due to improper wearing of the lap belt in case of a frontal collision. If the angle of the lap belt relative to the horizontal plane is too flat, the belt may slide over the iliac crest, thereby compressing the abdominal organs (see also Sect. 6.2). This also causes the lumbar spine to flex which can result in separation of the posterior elements of the spine, for instance, by ruptures of the supra- and interspinous ligaments. Furthermore, the spinal cord is stretched and might be injured.
Injuries of the soft tissue of the thoracolumbar spine are also often reported after automotive accidents. The soft tissues involved are the intervertebral discs, the various ligaments, the facet joints, the muscles and tendons attached to the vertebral column. A usual complaint of this type of injury is low-back pain. Incidents provoking this complaint are manifold, ranging from minor rear-end collisions to severe frontal impacts. In some cases the back pain is associated with disc rupture or disc bulge. However, a causal relationship between an impact and a rupture does usually not exist (King 2002). Disc ruptures are generally the result of a slow degenerative process.
4.3 Biomechanical Response and Tolerances
The mechanical performance of the human spine was subject to numerous volunteer, cadaver, animal and dummy tests. Experiments were conducted statically and dynamically (both with and without head impact) utilising different test set-ups (see also Sect. 2.5). Further, the use of so-called functional units is common in spine testing. Here a functional unit usually consists of a motion segment of two or three vertebrae. Tissue that is not of interest in the study performed (e.g. muscles) is dissected. Analysing the head-neck kinematics, some studies also use larger units that are made of a cadaver head and neck whereas the neck is fixed at its lower end and then mounted on a (mini-) sled. However, it has to be noted that the use of functional units can influence the kinematics significantly. This has to be considered when drawing conclusions from results obtained in such experiments. A thorough review on biomechanical experiments facilitating functional units under various loading conditions is provided by Jaumard et al. (2011).
Muscle activity can often not be simulated in experiments, because they are either removed (functional units) or without tonus (cadaver experiments). Only volunteer experiments offer the possibility to measure muscle activity to some extent. Tolerances to other spinal injuries, like for instance vertebral artery lesions, are difficult to assess because a physiological limit of the loading of the structure of interest cannot be defined properly.
Investigating the biomechanical response of the cervical spine, many studies still refer to and rely on tolerance levels based on volunteer and cadaver experiments that were performed in the late nineteen sixties and the early seventies, for instance by Mertz and Patrick (1967, 1971). Figure 4.14 shows a test set-up used to determine static properties of the neck.
Further, sled tests were conducted to account for the dynamic effects when loading the neck (Goldsmith and Ommaya 1984). Volunteer tests provided data up to the pain threshold, and cadaver tests extended the limits for serious injuries (Fig. 4.15).
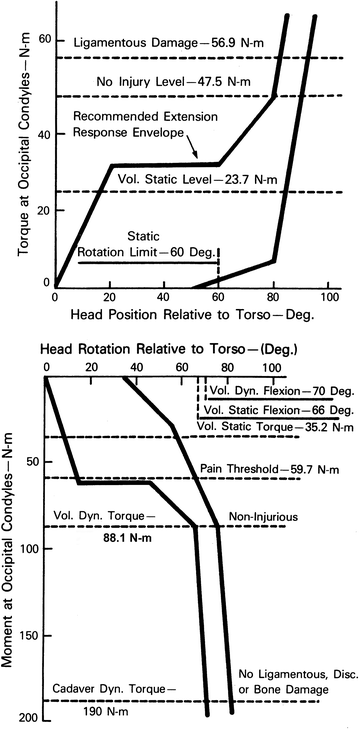
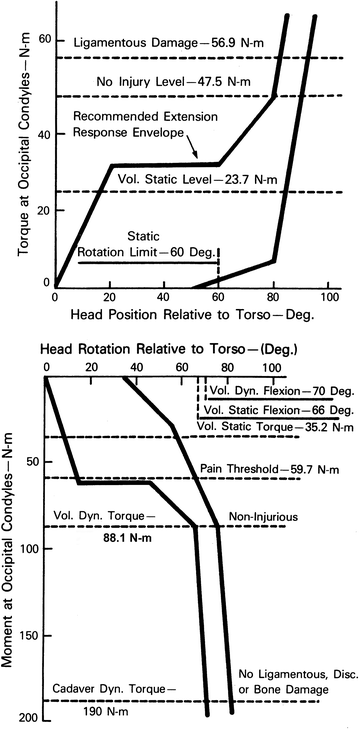
Fig. 4.15
Head-neck response envelope for extension (top) and flexion (bottom) as determined by Goldsmith and Ommaya (1984)
More recent studies investigated the relative motion of each vertebra in volunteer sled test experiments by using X-ray based techniques like cineradiography (e.g. Ono and Kaneoka 1997, 2001; Ono et al. 2006). This way the motion pattern of each vertebra could be assessed.
For the lumbar spine, fracture thresholds were determined by different experiments using functional spinal units. Compression fractures were observed for loads ranging from approximately 2–6 kN (e.g. Hutton and Adams 1982; Myklebust et al. 1983; Yoganandan et al. 1988; Myers et al. 1994; Belwadi and Yang 2008). Regulations in aviation safety require the maximum compression of the lumbar spine not to exceed 6672 (N = 1500 pounds). For more details see regulations CS 23–29 of the European Aviation Safety Agency (EASA 2013) or the corresponding U.S. standards, 14 CFR 23–29, of the Federal Aviation Agency (FAA 2013). Limits for frontal, rear-end and downward acceleration of the lumbar spine were proposed such that for impact durations of less than 100 ms, a 40 g threshold shall not be exceeded for well-restrained seated passengers (Viano 2001).
Table 4.3 summarises tolerance values of the cervical spine derived from the various experiments reported in the literature; however, due to differences in experimental techniques and test conditions, the data shows a considerable spread. Furthermore, one has always to bear in mind that tolerance, in addition to being a function of the loading environments, is related to a variety of factors including variability of the anatomical structures (e.g. in terms of geometry or properties such as bone density) and presence of degeneration. Hence, it is not surprising that differences for male and female tolerance values are observed. Nightingale et al. (1997) report significant differences when testing compressive failure. For compression tolerance McElhaney et al. (2002) conclude that for the cervical spine average forces of 1.68 and 3.03 kN result for females and males, respectively.
Table 4.3
Tolerance of the cervical spine to injury
Response measured | Test objects | Threshold criterion | Threshold value | Reference |
---|---|---|---|---|
Extension | Volunteers | No-injury (static) | 23.7 Nm | Goldsmith and Ommaya (1984) |
Pain | 47.3 Nm | Mertz and Patrick (1971) | ||
No-injury | 47.5 Nm | Goldsmith and Ommaya (1984) | ||
Cadavers | AIS2, ligamentous injury | 56.7 Nm | Goldsmith and Ommaya (1984) | |
Flexion | Volunteers | Pain | 59.4 Nm | Mertz and Patrick (1971) Goldsmith and Ommaya (1984) |
59.7 Nm | ||||
Maximum voluntary loading | 87.8 Nm | Mertz and Patrick (1971) Goldsmith and Ommaya (1984) | ||
88.1 Nm | ||||
Cadavers | AIS2 (no fractures) | 189 Nm | Mertz and Patrick (1971) Goldsmith and Ommaya (1984) | |
190 Nm | ||||
Compression | Cadavers | Bilateral facet dislocation | 1.72 kN | Myers et al. (1991) |
Compression injuries | 4.8–5.9 kN | Maiman et al. (1983) | ||
Tension | Volunteers | No-injury (static) | 1.1 kN | Mertz and Patrick (1971) |
Cadavers | Failure | 3.1 kN | Shea et al. (1991) | |
Shear (a-p) | Volunteers | No-injury | 845 N | Mertz and Patrick (1971) |
Cadavers | Irreversible damage | 2 kN | Goldsmith and Ommaya (1984) | |
Functional unit | (Odontoid) fractures | 1.5 kN | Doherty et al. (1993) | |
Functional unit | Ligament rupture | 824 N | Fielding et al. (1974) |
Further differences in the dynamic response of female and male volunteers (e.g. differences in head acceleration) were found in sled test experiments mimicking rear-end collisions (Linder et al. 2008; Carlsson et al. 2011, 2012a). Also geometric measures like a smaller circumference of the female neck might influence the kinematics in rear-end collisions resulting in higher angular acceleration (Dehner et al. 2007). For other factors such as muscle activation, it remains unclear whether (or how) they affect kinematics and injury outcome (e.g. Siegmund 2011).
Age, in contrast, does account for different biomechanical response and tolerance values (cf. e.g. Yoganandan and Pintar 2000; Yoganandan et al. 2002). Higher tolerance values for compression were, for example, reported by McElhaney et al. (2002) for young males compared to adults (tolerance ranges from 3.64 to 3.94 kN compared to the values for adults as mentioned above). Concerning the influence of spinal degeneration such as osteochondrosis, spondylosis, spondylarthrosis or spinal disc height reduction (without affection of the spinal cord) the literature is not conclusive. Related to neck pain, for instance, the literature does not report a clear link between degeneration and extent of clinical complaints (e.g. Meenen et al. 1994; Marchiori and Henderson 1996; Nykänen et al. 2007; Carroll et al. 2008).
4.4 Injury Criteria
In addition to the tolerance values for spine loading described in Sect. 4.3, several neck injury criteria are defined. Besides the rather simple load limits included in current regulations, more complex criteria are proposed, particularly regarding “whiplash” injury. Even criteria used to assess the same type of collision focus on different effects, thus the assessment of an impact based on one criterion may be useful; yet it might not be sufficient. Different criteria relate to different phases and, by their definition, emphasise different aspects of the occupant motion. Hence, neck injury criteria reveal important information which can be used to describe injury risk, but sometimes also allow conclusions about associated subjects like seat design or the injury mechanism.
In general, it is important to note that injury criteria are restricted to the conditions specified in their definitions. Application to other conditions, for example to other impact directions, has to be addressed carefully. Adjustments in the test procedure and/or the evaluation and the interpretation of the results obtained might be necessary. This holds, of course, also true for the choice of the anthropomorphic test devices. As described in Sect. 2.6.1, the designs of a dummy, and especially of a dummy neck, are very different. Therefore, when assessing neck injury by determination of neck loads and injury criteria, the influence of the dummy is always inherent (e.g. Muser et al. 2000, 2002; Bortenschlager et al. 2007). In this context it should be pointed out that there is currently no rear impact dummy available representing a female. Likewise specific injury criteria addressing the risk of female occupants are lacking, although the high incidence rate of soft tissue neck injuries in females is established. First steps towards female injury criteria and a female rear-end dummy were undertaking in recent years, but are not yet concluded (e.g. Schmitt et al. 2012; Carlsson et al. 2012b; Linder et al. 2013).
With respect to neck injury, the neck injury criteria NIC (Boström et al. 1996), Nij (Klinich et al. 1996; Kleinberger et al. 1998), and Nkm (Schmitt 2001; Schmitt et al. 2002a) are often used. While Nij was designed to detect severe neck injuries in frontal impact, the other two were developed with regard to soft tissue neck injuries in rear-end impacts. Work by Kullgren et al. (2003), Muser et al. (2003) and Davidsson and Kullgren (2011) show that NIC and Nkm correlate well with the risk of AIS1 neck injury sustained in rear-end collisions.
Consequently, these criteria are also included in the recently introduced “whiplash” related seat test procedures by Euro NCAP (see Sect. 2.6). Although the biomechanical foundation of this Euro NCAP assessment scheme is weak in several aspects (e.g. Bortenschlager et al. 2007; Farmer et al. 2008; Schmitt and Muser 2009), a correlation between such consumer tests and real-life outcome is not necessarily excluded; Kullgren et al. (2010) did in fact find a good correlation between Euro NCAP results and real-world crash and injury ratings. Further studies have also shown that cars fitted with more advanced whiplash protection systems performed better in dynamic testing and had a lower injury risk in real-life (e.g. Kullgren et al. 2007; Farmer et al. 2008).
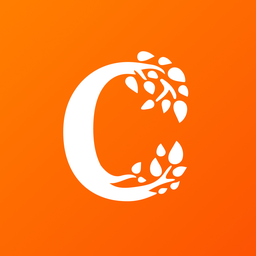
Full access? Get Clinical Tree
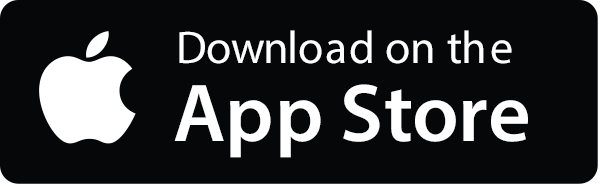
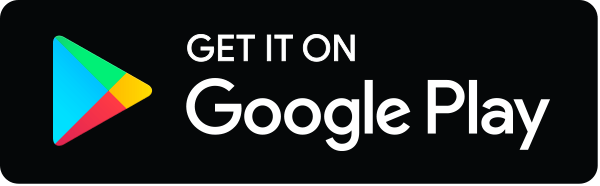