Fig. 17.1
Proximal stimulation with length-dependent neuropathy . Proximal stimulation (popliteal fossa) elicited tibial nerve SSEPs, whereas distal stimulation (ankle) did not in a patient with known diabetes but no documented history of neuropathy. This pattern suggests the presence of length-dependent diabetic neuropathy
Stimulation requirements are often increased in peripheral neuropathy and, as a rule, supramaximal stimulation of the peripheral nerve for SSEP acquisition improves the consistency of the responses. This makes establishing that a supramaximal level is present more important in the presence of neuropathy, and methods to elucidate supramaximal stimulation levels are discussed further in the “Technical Issues” section. It is further important to anticipate that SSEP latencies will be prolonged for patients with neuropathy and displayed time scales may need to be adjusted to avoid missing late-arriving SSEP waveform components. When stimulating more proximally, the relative latency of these components will be shorter than from a distal site, but may still be prolonged if the neuropathy is extensive.
Body habitus can present difficult challenges for the intraoperative neuromonitoring team. Those with little muscle mass may have a propensity for peripheral nerve compressions and additional padding may be needed to prevent or correct this risk. Conversely, patients with obesity suffer from increased rates of peripheral compression due to their increased body and limb weight and one must again be alert for these factors when evaluating SSEPs. Furthermore, with increased adipose tissue or peripheral edema, the distance from skin to target nerve for stimulation is increased, making it more difficult to supramaximally stimulate. For most stimulation sites, marginal increases in the distance from the site to the nerve can be overcome with simple increases in the stimulation intensity, assuming the underlying nerve remains normal. However, it is not uncommon that the underlying medical conditions that are associated with obesity or edema are also associated with peripheral nerve dysfunction. As a result, obesity or edema may complicate monitoring and more proximal stimulation sites, use of needle-stimulating electrodes as opposed to surface stimulation, or even use of longer-than-standard stimulating needles may be helpful.
Central Nervous System Dysfunction
Central nervous system pathophysiology also presents challenges to SSEP monitoring. Strokes, central demyelinating disorders such as multiple sclerosis, and other central disorders may have varying effects on SSEPs depending on the degree of central impairment. Central conduction delays, poor evoked waveforms, or absent evoked waveforms may result. In patients with demyelinating disease (e.g., multiple sclerosis), temperature management becomes particularly important as increased temperatures may impair neural transmission and therefore degrade signals in these patients (Uhthoff’s phenomenon). As such, it is important to document core temperatures and avoid relative hyperthermia in these patients.
Because scalp recording sites for SSEPs are a reflection of the electrode position relative to the brain tissue, any factor that alters this relationship may alter recordings. Scalp hematomas may dampen cortical response amplitudes; especially in patients with bleeding dyscrasia, a hematoma may even result from the placement of SSEP scalp recording needles. Similarly, subdural air, subdural hematomas/hygromas, or other tumors in this location have an even more profound effect and some of these may not be known to the intraoperative team at the time of surgery. When SSEP recordings point to dampening at a specific recording electrode rather than at all recording sites, one should consider a process possibly being present between the brain and scalp in addition to problems with the electrode itself. Similarly, a shift in the brain may occur after resection of a large tumor or when changing the position of the patient (supine to prone, supine to sitting position, or the reverse). Whereas the SSEP recording electrodes may stay in exactly the same position on the scalp, the underlying brain may shift slightly and therefore the relative orientation between brain and scalp may be altered and thereby affect SSEP signals.
During intracranial procedures, sterile field requirements may alter standard SSEP needle placements and thus changes in morphologies should be expected depending on the degree of displacement from standard electrode sites. In some cases, this alteration may be mitigated if the surgeon places sterile needles in the field. Neuronal migration disorders of cortical tissue (abnormal fetal migration of developing brain) or normal variation may also produce atypical SSEP cortical responses. For example, some patients will present with cortical waveforms following lower extremity SSEP stimulation that have a wider than typical N37 [1] distribution and a small or absent P37, thereby mimicking an upper extremity type of scalp distribution (“N37” morphology). Although this may be identified by its pattern if sufficient recording channels are utilized, verifying that the stimulation and recording electrodes are properly placed is also usually warranted for most atypical patterns (refer also to the section on technical issues in this chapter).
Motor-Evoked Potentials
Patient pathophysiology that affects SSEPs may similarly affect transcranial electrical MEPs . However, given the difference and variability in the central neural pathways that mediate these monitoring modalities, there is a complementary nature between SSEPs and MEPs and one may remain more reliable than the other in the face of preoperative neurologic dysfunction .
Peripheral Nervous System Disorders
As with SSEPs, radiculopathy may affect MEP signals and the degree to which this is manifest will depend on whether the affected myotome is relevant to the acquired motor signals. As muscles selected for MEPs may be innervated by different roots/nerves than those that mediate SSEPs, one can appreciate that MEPs and SSEPs may be differentially affected (Fig. 17.2). Neuropathies may also affect motor signals and, with the common length-dependent form, commonly used distal muscles for MEPs (i.e., thenar, abductor hallucis) may be disproportionately affected. In addition, neuropathies may be predominantly either motor or sensory, which may also contribute to disparities between MEP and SSEP baselines.
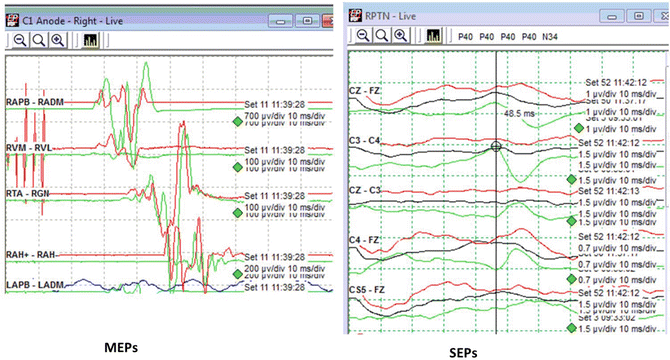
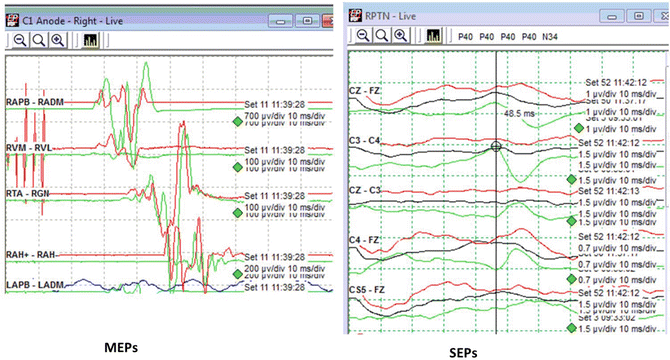
Fig. 17.2
New L5 radiculopathy affecting SSEP more than MEP. Right-sided MEPs (left panel) and SSEPs (right panel) taken at similar times during a lumbar surgery where a suspected root injury occurred. Note the clear disparity in that MEPs, including the posterior tibial nerve innervated abductor hallucis muscle (“AH”), show reproducibility to baseline levels, whereas SSEPs following right posterior tibial nerve stimulation shows dramatic and sustained decrease (red and black traces) from that of baseline (green trace)
Strategies are limited to address these issues for MEPs, but one should be aware of the surgical level of importance. Muscles used for recordings can be chosen outside of weak myotomes . Likewise, more proximal muscles can be chosen if significant distal motor neuropathy exists, although often proximal muscles are not as reliably monitored for MEPs. If a procedure involves high cervical levels (e.g., C3–4), deltoid muscles (C5 root) may well serve an important role in monitoring even if a severe neuropathy is present that precludes or limits MEPs in distal musculature. Given their reliance on conduction restricted to central white matter, D-wave recordings avoid peripheral nerve issues entirely, much in the same way they avoid anesthetic and neuromuscular junction issues.
Central Nervous System Dysfunction
Motor-evoked potentials are highly sensitive to a new myelopathy occurring during a surgical procedure, which leads, in part, to their utility in neuromonitoring. Unfortunately, sensitivity to a pre-existing myelopathy is similarly high, presenting a particular challenge for acquiring MEPs in that setting with marginal or absent signals often being the result. When this occurs, ideal anesthetic conditions as well as meticulous placement and adjustment of stimulating electrodes may be required for monitorable MEPs to be present. In addition, facilitating techniques may be required to increase the chance of eliciting a response or to maintain a response in the face of changing conditions or anesthetic fade during a procedure. These facilitating techniques are further discussed in the “Technical Issues” section below under the “Poor signals” subheading.
Electromyography and Nerve Conduction Studies
Electromyography (EMG ) monitoring and pedicle screw stimulation testing is reliant on an intact motor unit, and anything that disrupts the motoneuron, root, nerve, neuromuscular junction, or muscle may have an effect. The presence of applicable disease states and particularly weakness in target muscles should warn the neurophysiology team of possible limitations in the use of these modalities. (For more information on nerve conduction studies, see Chap. 38, “Surgery in the Peripheral Nervous System”).
Radiculopathy or neuropathy may greatly reduce the sensitivity of EMG monitoring as mechanical perturbation of roots or nerves may not be as readily reflected at the muscle in these conditions. The location and duration of the lesion are influential in how these effects are demonstrated. An acute lesion leaves the distal nerve functionally intact, which can be dangerously misleading if the nerve is stimulated distal to the lesion yielding a robust compound muscle action potential (CMAP) and giving the impression it is functionally intact (e.g., in facial nerve testing). Thus, testing as proximal as possible is preferred to assure functional integrity of the nerve. With more chronic axonal lesions, Wallerian degeneration proceeds within a week and the distal nerve will also show dysfunction as well, although in rare cases the distal nerve may remain functional in a nonfunctioning nerve (neurapraxic conduction block).
Radiculopathy/neuropathy may also significantly impact testing that relies on stimulation thresholds such as pedicle screw electrical testing. In the normal setting, a high stimulation threshold suggests that the screw is well-insulated by bone and thus is appropriately positioned. However, falsely elevated thresholds due to nerve dysfunction may occur during pedicle screw testing in the presence of radiculopathy/neuropathy and, as such, test results involving weak myotomes should be interpreted with caution. If possible, direct proximal root stimulation may help determine if a root has a stimulation threshold in the normal range (e.g., <4 mA using typical stimulation parameters). It may also be helpful to examine relative thresholds if multiple screws are evaluated and the finding of a threshold that is much lower than companion screws should alert suspicion and receive additional evaluation for malpositioning from the surgeon. Similarly, for free run EMG monitoring, it should be kept in mind that sensitivity may be affected depending on the muscles tested and severity/distribution of the radiculopathy/neuropathy.
Pre-existing neuromuscular junction disorders may also influence EMG testing. Depending on the type of disorder, measures of neuromuscular blockade may also yield unreliable results and the response to pharmacologic neuromuscular blockade may be highly exaggerated and prolonged.
Electroencephalography
Electroencephalography (EEG ) is typically monitored either by scalp electrodes and/or by direct cortical recordings (electrocorticography) during intraoperative neuromonitoring. EEG may be useful for such intracranial surgeries as aneurysmal or other vascular repair, brain mass excisions, and in epileptic foci excision. It is also useful in extracranial surgeries like carotid endarterectomies to assess for hemispheric ischemia.
Unprocessed EEG is also useful in identifying the deep anesthetic state that exhibits a burst-suppression pattern (Fig. 17.3). This state is easy to identify and may be useful as a surrogate marker of other synaptic suppression affecting neuromonitoring signals (e.g., those related to MEPs) and the deep cortical anesthetic state supplies information to the anesthesia team that they may use to titrate anesthetics. On the other hand, if the goal is to assess through the entire range of anesthetic depth, EEG requires digital processing techniques (e.g., Bispectral Index [BIS] monitor ) that are often imperfect and fall outside the usual realm of the neuromonitoring team (see Chap. 11, “Clinical Application of Raw and Processed EEG”).
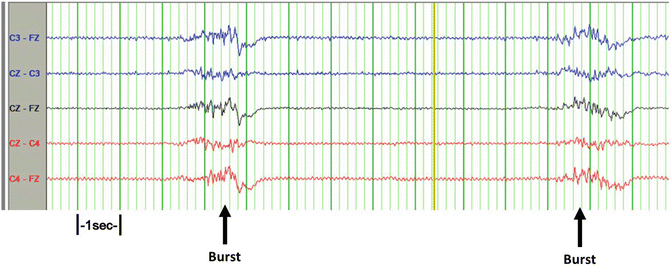
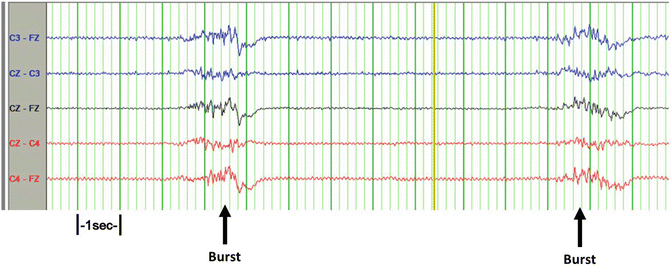
Fig. 17.3
Burst suppression pattern on the EEG. A burst suppression pattern follows a bolus of propofol on this limited EEG montage
Those anatomic barriers that insulate or alter SSEP recording electrodes (as discussed previously) can have the same inhibitory effect on obtaining EEG traces. In particular, hygromas, subdural hematomas, and scalp hematomas are examples of fluid spaces that will insulate scalp needles from underlying cerebral generators of EEG. These and other preoperative abnormalities such as focal attenuation, epileptiform discharges, or rhythmic slowing are likely to be present prior to surgery, making preoperative or preincision EEG a helpful reference to distinguish between these pre-existing displays and new findings (Fig. 17.4).
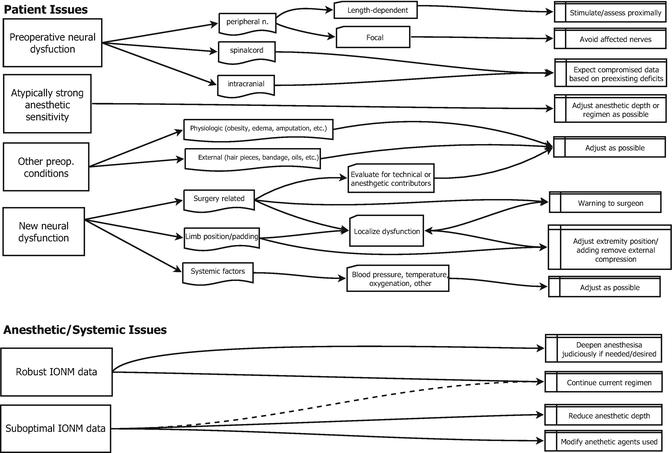
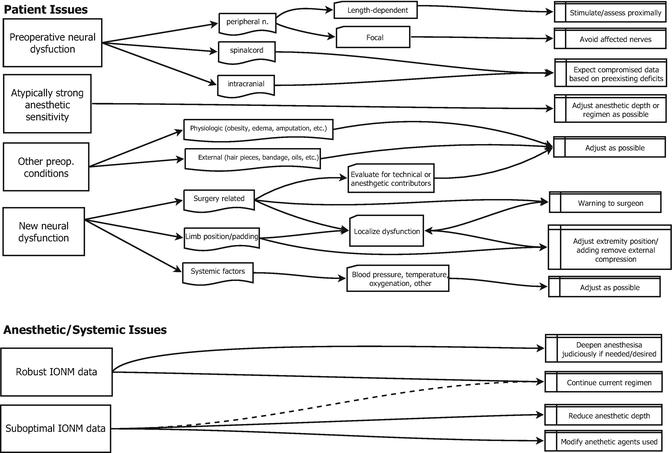
Fig. 17.4
Patient anesthesia chart
Anesthetic and Systemic Effects
The goals of anesthesia, analgesia, and amnesia may at times be in direct opposition to obtaining robust neurophysiologic data . Those neurophysiologic functions requiring more synapses are in turn typically the first to be blunted by anesthesia (e.g., conscious acts) and differential effects are noted within neurologic pathways utilized by common neuromonitoring tests (e.g., MEPs affected to higher degree than SSEPs). Although a bell curve may generally exist for the average anesthetic affect across patients, there is wide variation for an individual patient’s responses.
A number of systemic variables may also impact neuromonitoring data both directly and through the patient’s reaction to an anesthetic. Temperature, pH, blood pressure, hematocrit, oxygenation, CO 2 levels, blood loss, fluid status, and other factors can impact monitoring signals, which can dramatically change intraoperatively [2]. Stability of anesthetic and systemic parameters improves the stability of neuromonitoring data and allows for a clearer interpretation. However, the reality is that some degree of variation is inevitable and wide degrees of change may be unavoidable. Understanding the impact that anesthetic/systemic variations have on neuromonitoring data and the ability to differentiate this from new neural dysfunction is part of the art of intraoperative neuromonitoring. For more details, see Chap. 19, “Anesthesia Management and Intraoperative Electrophysiological Monitoring.”
Somatosensory-Evoked Potentials
Because anesthetics produce their greatest effect on pathways with the greatest number of synapses, SSEPs recorded from cortical generators are affected more than recordings from subcortical sites. As seen in Fig. 17.5, the A1-Fpz subcortical channel recordings are unchanged relative to baseline even though the cortical channel recordings have been greatly diminished with increases in anesthesia. Because the operation in this case was an orthopedic spine case, the stability of the subcortical response helps to provide reassurance that conduction is proceeding normally across the surgical levels despite the large changes from anesthesia. The largest subcortical response typically is generated in a Cerv5-Fpz channel, which adds a small N13 cervical component to what is primarily positive medullary activity (P13, P14) recorded in the Fpz electrode. Additionally, a pure medullary response is purposefully recorded in cervical level cases by foregoing the Cerv5 electrode for one above the neck such as from the ear (A1 in Fig. 17.5).
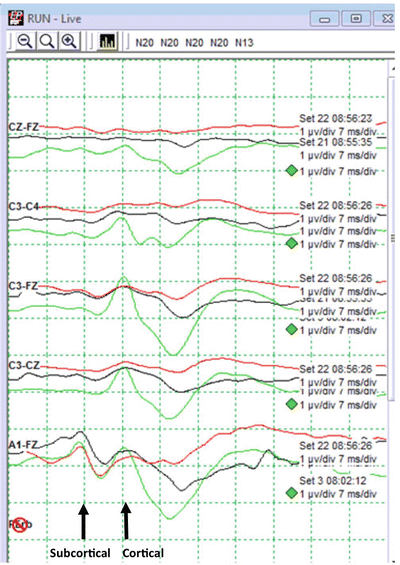
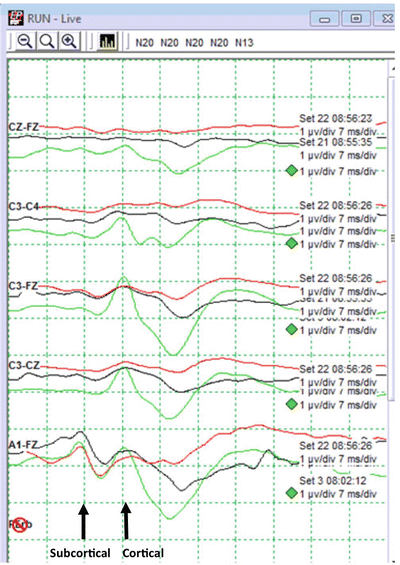
Fig. 17.5
Anesthetic suppression of cortical SSEP responses. Right ulnar SSEP recordings were obtained during a cervical spine fusion using four cortical (Cz-Fz, C3-C4, C3-Fz, C3-Cz) and one subcortical channel (A1-Fz). The green traces are baseline responses, red traces are current traces, and black traces are the previous set. After an increase in anesthetic gas, the subcortical response is stable, whereas all cortical traces are blunted and delayed compared with baseline (a similar pattern was present in left ulnar recordings at the time)
Use of the subcortical response to identify anesthetic effects in lower extremity SSEPs may be more problematic because these subcortical signals are notoriously more difficult to obtain as compared with those from the upper extremities. Thus, for thoracolumbar procedures, when a subcortical response is not available to aid interpretation, one may have to rely on cortical responses that are inherently more affected by anesthetics. In this situation, stability of the upper extremity subcortical signals in the face of both upper and lower extremity cortical signal decline can still lead to a conclusion that an anesthetic/systemic effect is at play.
Other limiting factors for the acquisition of a subcortical response are its particular susceptibility to electrical noise due to the long interelectrode distances and the presence of myogenic artifact due to the proximity of recording electrodes to muscle. As a result, these signals are commonly degraded when a patient is under relatively light anesthetic and is not under neuromuscular blockade. If these are the causes, the noise may be abated with increased anesthetics and/or delivery of neuromuscular blockade, although these may often not be desirable for other reasons. Alternatively, narcotics and other intravenous agents will typically have lesser effect on the cortical and subcortical components of the signals while still effectively mitigating EMG-induced artifact.
Although stable subcortical responses may confirm adequate dorsal column function across the surgical levels in spine surgeries, intracranial neurologic dysfunction remains possible despite a preserved subcortical response. A not uncommon example exists in the case of anterior cervical procedures where the placement of retractors may impinge on a carotid artery. If adequate collateral flow is not present, hemispheric ischemia may result that will affect cortical responses from the contralateral upper extremity while leaving the associated subcortical response unaffected. Thus, in conditions when cortical responses are diminished and one has to rely on subcortical SSEP responses alone, a change due to carotid retraction could readily be missed.
Increased anesthetics, decreased oxygenation or hematocrit, decreased perfusion pressures, and perturbed pH may all similarly affect SSEPs with a preferential effect on cortical SSEPs. Although one may expect globally similar or at least symmetric bilateral effects on cortical responses with increases of anesthesia, practical experience not uncommonly demonstrates quite asymmetric changes due to anesthetic alterations. These often appear accentuated when baseline asymmetries already exist and patterns may emerge that greatly increase the chance that changes are mistakenly interpreted as arising from a surgical cause.
Motor- Evoked Potentials
Anesthetic agents and systemic changes may have profound and variable effects on MEPs. Inhalational agents potently suppress the ability to activate motoneurons. As a result, propofol, supplemented at times with ketamine and/or narcotic, has become a widely used agent and its use is more conducive to acquiring MEPs compared with the use of inhalational agents at similar degrees of anesthetic depth. However, it should be kept in mind that any anesthetic agent, whether intravenous or inhalational, is capable of inhibiting MEPs and high-dose propofol can also dramatically suppress MEPs [3]. Measuring the blood’s concentration of intravenous (IV) agents is rarely practical, and physiologic measures such as using a BIS EEG-type monitor have been imperfectly reliable. Simpler EEG measures, as noted previously (Fig. 17.3), can identify burst suppression indicating a deep cortical level of anesthesia. This may be used as a guide to the anesthesia team that anesthetics are becoming excessive as a deep anesthetic state is clearly reached with burst suppression. At that point, a reduction in anesthetic delivery might be considered and any further increase in anesthetics would be expected to further suppress neurophysiologic responses, to increase the incidence of propofol infusion syndrome [4] and to increase the likelihood of long wake-up times at the end of the procedure. In addition, bolus dosing of propofol may yield wide swings in MEP suppression, leading to a difficult interpretative environment for the monitoring team.
For practical purposes, some mixture of inhalational gases and IV anesthetics are commonly used in procedures that use MEPs. However, patients exhibit vastly different sensitivities to inhalational agents in terms of the suppression of their MEPs. For patients who are particularly sensitive or for patients who are myelopathic or have other neurologic impairment, MEP signals may not be recordable. When this occurs in the setting of a mixed inhalational and intravenous anesthetic technique, a subsequent shift toward intravenous and away from inhalational agents can improve the likelihood of obtaining useful MEPs in these cases.
When poor or absent MEPs are encountered, a number of steps focused on improving the stimulation delivery may be taken in parallel with efforts to improve the anesthetic environment. These are discussed previously and also in the “Technical Issues” section below under the “Poor signals” subheading.
The level of neuromuscular blockade is obviously an important additional factor in the acquisition of MEPs. However, unlike anesthetic agents, of which the action is on already difficult to activate central synapses, the action of neuromuscular blockade is more predictably linear on the amplitude of the MEP. That being said, an understanding of the state of this blockade is important and high levels of blockade will prevent MEP acquisition. We find it helpful to utilize train-of-four measures in each limb so as to provide the most accurate assessment of the systemic level of blockade and to assess for focal variability. MEPs are also sensitive to other systemic parameters such as blood pressure, pH, oxygenation levels, and hematocrit. It is not uncommon that MEPs can slowly diminish in their response over the course of a procedure and this is likely a combination of anesthetic effects and the inevitable changes in blood composition that occur during surgery such as blood loss. Resultant decreases in hematocrit and thus oxygenation with concomitant pH perturbations are all thwarting factors to the acquisition of MEPs. An additional adverse systemic factor that is frequently overlooked when attempting to acquire MEPs is rising core temperature with the use of body warmers. Mild hypothermia may actually improve MEPs [5], while extremes of temperature in either direction will impair MEP monitoring. As with SSEPs, all of these systemic parameters may exacerbate the effects of anesthetics and it is common to see these culminating effects with time; especially in longer surgical procedures.
Electromyography
EMG monitoring is relatively immune to the effects of anesthetic agents; appropriate monitoring typically requires only that neuromuscular junction functioning be intact. Inhalational and intravenous agents used for anesthesia do not have a clinically evident effect on this junction. However, paralytic agents are commonly used, and by definition, may have a profound effect on EMG activity. Sensitivity to the effects of these agents varies greatly across patients as does the recovery of neuromuscular junction function. Therefore, the effect of this dosing is not precisely predicted and instead must be measured at the muscle. For pedicle screw testing, a T1 % (amplitude of an evoked supramaximal abductor pollicis brevis [APB] muscle response as compared with preanesthetic baseline) of over 20 % has a minimal effect on threshold testing [6], which translates to the more easily electrically measured train-of-four ratio in the gastrocnemius of 35 % (unpublished data). Visually assessed mechanical measures of ulnar nerve “twitches” has wide inter-rater variability, although, if used, this level of blockade usually corresponds to three or four twitches out of four. Levels of partial blockade that do not affect the sensitivity of free-running EMG are not well-defined, but are likely not clinically impaired at those parameters discussed before. When in doubt and when possible, erring on the side of less blockade is preferred.
Electroencephalography
Certain anesthetics may have a more pronounced effect on EEG than others, but sufficient concentration of any anesthetic may alter EEG. Boluses of intravenous anesthetics in particular may also influence EEG greater than say SSEPs; if there is a sudden global suppression in EEG while SSEPs remain stable, an anesthetic-related contributor is the usual cause (see Fig. 17.3).
Differentiation of effects on our signals from systemic factors versus from new neurologic dysfunction is not always clear cut and some alterations in systemic patient physiology may contribute to new focal neurologic dysfunction. For example, relative hypotension in isolation may affect some signals without expectation of adverse sequelae in most cases. However, if surgical steps result in tissue that cannot tolerate any decrease in perfusion, even mild relative hypotension could cause a critical threshold for tissue ischemia to be surpassed. As such, one often needs to simultaneously take action related to possible new neurologic dysfunction while addressing other factors that may be obscuring the ability to correctly identify potential new dysfunction.
Technical Issues
“Technical issues” is a broad term that concerns the technique used to acquire signals. As noted in the Introduction, these issues have their roots in good basic technique, and a solid working knowledge of neuromonitoring is assumed. Beyond basic technique, we hope to address further coping strategies in response to the patient and anesthesia challenges discussed previously, the sometimes electrically hostile operating room environment, equipment failure, and unintended deviations from usual methods.
As in all areas of signal optimization, a rational step-wise approach to understanding, localizing, and addressing the specific issues at hand will provide the best route to timely and effective optimization. In contrast, inexperienced persons may try steps “because they worked before” (often for a different problem) or in an otherwise illogical way. A moment of thought in these situations will often save valuable time, allowing redirection of attention to other optimization or even the monitoring itself. One extreme example is someone who wanted to “replace all the electrodes” when encountering a high-amplitude noise problem, but instead, with some quick investigation, showed that this was an external noise source (unplugging the bed solved the problem). Once issues are identified as technically related (as opposed to or in addition to patient/anesthesia-related issues), our goal is to further break down the issues until we come to a specific answer (Fig. 17.6).
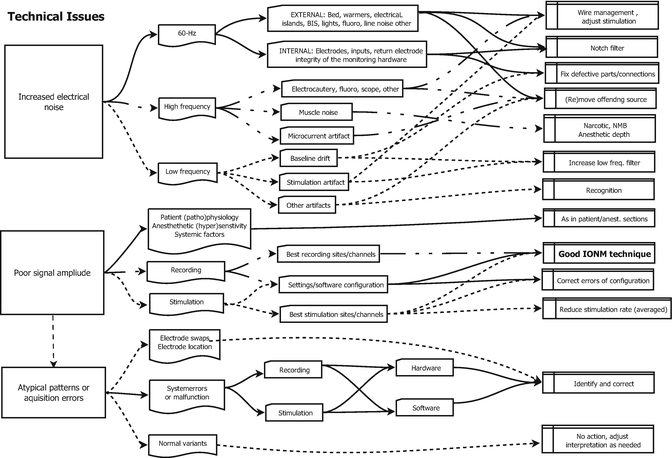
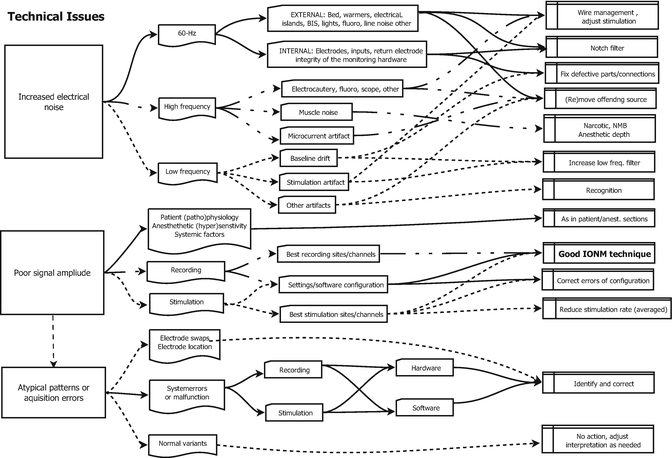
Fig. 17.6
Technical chart
For technical issues, we first decide whether the problem fits into one of three broad areas: increased electrical noise, poor signal amplitude, or atypical patterns of signal response. One will quickly recognize that the first two categories are the two components of the SNR, and deciding the root cause of a poor signal is usually deciding whether the problem is primarily increased noise, decreased signal, or both. This distinction hinges on both an understanding of the usual levels of background noise and the usual response characteristics of target signals. If we encounter a poor SNR and the amplitude of electrical noise exceeds our expectation for typically sized signals, then we’d expect that electrical noise is our primary issue. If, on the other hand, the level of electrical noise is below the range of typical signals, then we expect that low signal is the primary cause. This may appear trivial, but some common errors demonstrate that this thought process is not universally applied. For example, most of us have probably encountered a situation in which the sensitivity for recording has been increased to a point that universally present intrinsic noise is displayed prominently and the absent signals are described as “noisy.” Conversely, with high noise levels, recording sensitivity may be decreased to make the baseline superficially appear to have a reasonable noise level when, in fact, embedded signals would never be recognizable. The key to avoiding these errors is to have an understanding of expected amplitude and latency of the target signal. If one knows their target values, they will adjust their settings to those values rather than to a potentially deceiving “look” and the differentiation between noise and signal issues should be much easier.
The third main category for technical issues primarily deals with errors in the configuration of our monitoring systems or electrodes. This may be due to failure to correctly configure or connect components or it may be due to breakage of the equipment. When these “errors” occur, signals may be present in unexpected patterns/morphology, but possibly with reasonable recording fidelity. At other times, select signals will be absent in patterns that do not make physiologic sense or signals may be completely and unexpectedly absent but possibly with clues such as absence of stimulation artifact.
As is apparent, there is overlap between the “poor signal” category and the signal acquisition error category. In fact, one might logically consider this third category as a subdivision of the poor signal category. For discussion and thought organization purposes regarding the signal acquisition error category, we will focus on frank errors or breakage, whereas in the prior category, we will focus on reasonable but potentially suboptimal aspects of either the stimulation or the recording systems.
Increased Electrical Noise
Electrical noise is a constant accompaniment to neuromonitoring and some level of background noise is omnipresent and must be tolerated. However, a number of routine strategies are used to limit ubiquitous noise such as common mode rejection amplifiers, signal averaging techniques, and electrical filtering. When, despite these measures, the amplitude of electrical noise increases to the point that it adds variability to or overwhelms the recorded signals, it may interfere with identification and interpretation of neuromonitoring data.
When feasible, the best and most common strategy for dealing with noise is to remove or mitigate (by changing the source’s location or the location of recording equipment/wires) the offending noise generator. In order to remove noise, the source must first be identified. One possible strategy is to note where the noise amplitude is the greatest, and if our signals point to a specific area of the body, we can look in that area first. Unfortunately, a clear focality to noise is not always obvious, so we also characterize the frequency of the intruding noise, which may yield clues as to its source (Table 17.1).
Table 17.1
Common sources of elctrical noise
External sources of 60-Hz noise | Internal sources of 60-Hz noise |
1. The operative bed | 1. Poor/partial electrode contact with the body |
2. Fluid warmers | 2. A damaged electrode |
3. Body warmers | 3. Poor connection of the electrode to the recording system |
4. Electrical islands, extension cords | 4. Dysfunctional jackbox, associated cable, or their connection |
5. BIS monitors | 5. Dysfunction amplifier |
6. Fluorescent lights | 6. General current leak in the system |
7. Fluoroscopy unit | |
8. Other electrical device in the vicinity | |
9. Power line noise (e.g. nearby heavy equipment use) | |
High-frequency noise | Low-frequency noise |
1. Muscle artifact | 1. Stimulation artifact |
2. Microcurrent artifact | 2. Movement artifact |
3. Electrocautery activation | 3. High-amplitude noise transients “intrusions” |
4. Electrocautery return pad (constant impedance testing) | 4. “Baseline drift” from poor electrode contact
![]() Full access? Get Clinical Tree![]() ![]() ![]() |