Introduction
The first moment of healthy extrauterine life is usually heralded by a deep breath followed by a cry. Within a few breaths, the color of the infant changes from a mottled dusky blue to a rosy hue and the infant is generally alert, looking around at his new environs. Once he is swaddled and brought to his mother, he may instinctively root and suck even though the mother is not yet able to nourish him. The physiologic changes that occur in the first few minutes of life are astounding. During development, the fetus develops an organ physiology that was adapted to the low-oxygen environment of the maternal circulation and then the first breath of extrauterine life initiates a process of physiologically altering his circulation and respiration. In order to care for neonates during the perioperative period, it is important to understand the physiologic changes that occur during the first few hours, days, and months of life.
Cardiac
In utero, oxygenated placental blood is divided to pass through either the liver or to the inferior vena cava via the ductus venosus. This oxygenated blood from the inferior vena cave preferentially streams across the right atrium through the foramen ovale into the left atrium. This blood passes through the left ventricle and aorta to supply the myocardium, head, and upper torso. Deoxygenated blood returns to the right atrium via the superior and inferior vena cava and is pumped out into the pulmonary arteries via the right ventricle [1]. About 11 percent of this blood is distributed to the highly resistant pulmonary vascular bed and 89 percent is distributed to the aorta via the ductus arteriosus [2]. Aortic blood then divides to supply the lower extremities and to return to the placenta via the umbilical arteries to be reoxygenated.
Immediately after delivery, with the first breath of life, the expansion of the lungs causes the pulmonary vascular resistance to drop. Concurrently, the umbilical vessels begin to narrow due to traction and the increased oxygen levels in the umbilical artery from the infant breathing the room air. Once these vessels are fully constricted or clamped during delivery, the vascular resistance of the systemic circulation is markedly increased. The result of this is less blood flow through the ductus venosus to the inferior vena cava. The decrease in pulmonary vascular resistance leads to more blood flow to the lungs and higher flows into the left atrium. Thus, the right- and left-sided cardiac pressures begin to reverse, with the left atrium developing higher pressures, which causes the foramen ovale to functionally close. The flow across the ductus arteriosus becomes left-to-right as the pulmonary artery pressure decreases and the system vascular pressure increases. Normally the combination of decreased circulating prostaglandin PGE2 and the increased oxygen levels within the duct lead to functional closure within 2–3 days in greater than 99 percent of term infants [3]. Complete closure does not occur normally until 4–8 weeks, which means that under certain circumstances the ductus arteriosus can reopen. If the vascular resistances of the pulmonary circuit become elevated during this period, it is possible for the foramen ovale to act as a right-to-left shunt, as it did during fetal life. This condition is known as persistent pulmonary hypertension of the newborn and can be exacerbated by hypoxia, acidemia, or primary structural cardiac diseases. Figures 1.1 and 1.2 offer an overview of the circulatory system.
Figure 1.1 The fetal circulatory system (from Rice University’s OpenStax course in Anatomy & Physiology under CCC license 4.0 http://cnx.org/content/col11496/1.6/).
Figure 1.2 Fetal blood circulation © BSIP/UIG.
Neonatal Heart
In a full-term infant, the neonatal cardiac output at 200 ml–1 kg–1 min–1 or greater is roughly double that of adults to meet the metabolic demands of eating, breathing, thermogenesis, and growing [4,5]. Because the myocardium is immature at birth, with a reduced, chaotic arrangement of myofibrils, there is limited compliance at birth [5]. Although filling pressures can increase stroke volume to a small degree, functionally the neonatal heart is primarily dependent on an increase in heart rate to increase cardiac output. At birth the ECG reflects the right-sided dominance of the fetal heart with right axis deviation and R-wave dominance in lead V1 and S-wave dominance in lead V6. After the left ventricle grows and hypertrophies, the ECG assumes the adult configuration of left-sided dominance by about six months of age [5]. Mild congestive heart failure in young infants is often heralded by sweatiness, tachycardia, tachypnea, difficulties in feeding, and may be missed during a preoperative examination. In cases of moderate to severe congestive failure there will be signs of acidosis, hypothermia, and oliguria. Management consists of diagnosing the underlying physiology, fluid restriction, diuretics, and inotropic agents.
Normal heart rates at birth at rest vary between 104 and 156 (mean 130 + 13 mmHg). The first day of life, the mean systolic blood pressure is 70 mmHg, which rapidly increases over the next three days to 77 mmHg [6]. Mean blood pressure rises by 15 percent over the first month of life [7].
Congenital Cardiac Defects
The most common birth defect in neonates is congenital heart disease (CDH) with a prevalence of between 6 and 13 per 1000 live births [8]. It is 2–3 times more common in premature births compared with full-term births [9]. Critical CDH is defined as lesions requiring surgery or catheterizations within the first year of life, and constitute 15 percent of CDH at birth [10,11]. Approximately 30 percent of critical CDH is diagnosed after initial hospital discharge because the ductus arteriosus is still functioning, masking symptomatology [10,11]. The most common lesions involved include coarctation of the aorta, interrupted aortic arch, aortic stenosis, transposition of the great vessels, and hypoplastic left heart syndrome. Some nondependent duct lesions associated with mild cyanosis such as truncus arteriosus, Tetralogy of Fallot, and total anomalous venous return can be missed during the perinatal period. Missed critical CDH has a high mortality rate, with more than half of patients dying before surgical repair was done [12]. Presentation usually occurs when the ductus arteriosus begins to close and the infants manifest either shock or cyanosis. Careful physical examination of term newborns scheduled for surgery is important, but may not detect all infants with critical CDH. A cardiac murmur is present in many patients with CDH, but in approximately 44 percent of murmur detections in a healthy newborn there is no structural heart disease [13]. A careful physical examination along with a screening pulse oximetry test should be carried out for every preoperative patient. Attention should be paid to abnormal heart rates, precordial activity, S2 splitting, other heart sounds, murmurs, peripheral pulses, and cyanosis. In patients with cyanosis, a hyperoxia test can be done to help discern cardiac from noncardiac causes of decreased oxygen desaturation. In addition to the hyperoxia test, pulse oximeters can be placed both preductally (right hand) and postductally (left limb) to determine whether there is differential saturations. In medical centers with access to echography, a cardiac echo can rapidly determine the structural integrity of the heart.
Respiratory
The process of extrauterine breathing occurs with fluid being squeezed from the lung during vaginal delivery. The first breath is characterized by high inspiratory negative pressures to overcome the airway resistance of residual fluid in the airways, and pulmonary collapse. With alveolar expansion, the alveolar size increases and the wall tension of the alveolus decreases. Alveolar collapse between breaths is limited by a coating of surfactant that maintains surface tension within the alveolus. Type II pneumocytes are responsible for surfactant production and begin to differentiate at 24 weeks of gestation; however, surfactant synthesis for appropriate pulmonary function is not adequate until 34–36 weeks of gestation [5]. Once oxygenated blood reaches the pulmonary and central circulation, the pulmonary vascular resistance drops and facilitates the transition from fetal to adult circulation.
Neonatal lung mechanics put young infants at a disadvantage compared with older children and adults. The chest wall is exceedingly compliant and the lungs themselves relatively noncompliant; both of which can facilitate lung collapse. In fact, the closing capacity for neonates exceeds functional residual capacity. Neonates compensate for this by producing auto positive end-expiratory pressure (auto-PEEP) by partial closure of the vocal cords and breathing through the high-resistance nasal passages. The horizontal nature of the ribs and the relatively flat diaphragm make the work of breathing inefficient and increases in minute ventilation are achieved by increasing respiratory rate. Also, infants have a relatively low functional residual capacity compared with adults. Any surgical pathology that decreases the compliance of the abdomen will necessarily affect the ability of the neonate to adequately ventilate. During the first day of life, ventilation flow mismatch accounts for up to 24 percent of cardiac output. This fraction decreases to 10 percent by one week of life [14]. In addition to the disadvantageous lung mechanics, neonates consume twice as much oxygen per kilogram as adults, thus their alveolar ventilation needs to be roughly double that of adults. Because of all these factors, modest decreases in either ventilation or fraction of inspired oxygen (FiO2) will incline neonates to develop hypoxia.
The ventilatory centers of respiration in the brain at birth are not fully developed, and responses to hypoxia are immature in the term neonate. The peripheral chemoreceptors do not respond to hypoxia during the first 48 hours of life because they are effectively suppressed by the rise in PaO2 following delivery and need to be reset [15]. This effect is exacerbated in infants resuscitated with 100 percent O2 in the delivery room [16]. The feedback mechanisms by which the peripheral chemoreceptors send messages to the central centers of ventilation are also not mature, leading to unstable respiratory control mechanisms, especially in sick, unstable neonates.
Apnea and bradycardia is one of the nonspecific signs that a neonate is suffering from stress such as hypoxemia, sepsis, or hypothermia. This apnea can lead to an unstable metabolic state that further increases the risk of apnea and hypoxia. Apnea, which is generally defined as a cessation of breathing for longer than 15 seconds, can also result from airway obstruction, especially in those neonates with congenital abnormalities of the head and neck region or neonates that have been sedated with anesthetic drugs [17]. It is very common for term infants after a general anesthetic to exhibit periodic breathing, which is a breathing pattern in which the tidal volumes become shallower and shallower to the point of brief cessation, and then become deeper again. This pattern is repeated and generally does not lead to oxygen desaturations. Some studies – but not all – have shown an association between anemia and an increased risk of postoperative apnea [18].
Hematologic
At birth, 50–95 percent of the hemoglobin in an infant is fetal hemoglobin, which has an increased attraction for oxygen compared to adult hemoglobin. Fetal hemoglobin is made up of two alpha chains and two gamma chains, and is gradually replaced by hemoglobin A, which is made up of two alpha chains and two beta chains, by the time the infant is six months old. The fetus lives in a relatively hypoxic zone compared with the maternal environment and compensates for this with a much higher hemoglobin concentration of 19.3 g dl–1. Within one week of birth in term infants, hemoglobin levels begin to drop and reach a nadir of about 9–11 g dl–1 at about 8–12 weeks of life [19]. Anemia can occur at birth as a result of blood loss before, after, or during delivery. Feto-maternal or twin–twin transfusion, placental abruption, or delayed cord clamping can cause increased blood loss. Decreased erythrocyte production can occur as a result of iron deficiency, or chronic infection. Decreased red blood cell destruction can be caused by Rh and autoimmune hemolytic disease, enzyme abnormalities such as G6 PD, membranopathies such as spherocytosis and elliptocytosis, and hemoglobinopathies such as sickle cell disease, thalassemia, and hemoglobin C disease.
Term infants can also be born with polycythemia, which can lead to a hyperviscous state and complications when the hematocrit is greater than 65 percent [20]. Maternal uterine insufficiency can lead to placental hypoxic state in which the fetus responds with increased red cell production. Postnatally polycythemia can cause decreased blood flow to vital organs including the brain, heart, and lungs, increased bilirubin production, and is associated with hypoglycemia. Treatment is a partial exchange transfusion.
The coagulation profile of neonates also differs from adults. Many of the proteins needed for coagulation are diminished in newborns, including factors II, VII, IX, X, XI, XII; others that promote fibrinolysis are increased, such as thrombomodulin, tPA, and plasminogen activator inhibitor-1 [21,22]. These pro-bleeding tendencies are balanced by an alteration in some procoagulant proteins such as an increase in Von Willibrand factor and a decrease in antithrombin, heparin cofactor II, alpha-2-macroglobulin, protein C, protein S, and plasminogen [22]. The end result is that neonates normally have a prolongation of their prothrombin time (PT), and activated partial thromboplastin time (aPTT), but a shortened bleeding time [21].
Newborns can be adversely affected by low vitamin K levels. Bleeding can occur because of a decrease of the vitamin K-dependent coagulation factors (II, VII, IX, X). Treatment includes prophylactic vitamin K intramuscular injections and fresh frozen plasma transfusions in infants with symptomatic bleeding.
Early thrombocytopenia in the newborn is usually related to maternal–placental factors, and late thrombocytopenia is usually caused by excessive platelet consumption, such as seen in necrotizing enterocolitis and sepsis [23]. Thrombocytopenia is a risk factor for intraventricular hemorrhage even in term infants, so many centers administer platelet transfusions to infants whose levels are below 50 000.
Thermoregulation
Regulation of body temperature depends on a balance between heat loss and heat generated. Although thermogenesis is suppressed in utero, heat production is actually greater in the fetus than the mother so the heat gradient flows from the fetus to the mother. Humans are a precocial species in regards to thermogenesis, with responses capable of generating heat within minutes of birth. Stimulation of the preoptic chiasma/anterior hypothalamic nuclei from peripheral cutaneous receptors activate non-shivering thermogenesis by sympathetic norepinephrine-secreting nerve fibers that innervate brown adipose tissue and shivering thermogenesis through the posterior hypothalamic nucleus [24].
Hepatic
During fetal development, the umbilical vein brings blood to the liver. Between 20 to 30 percent of this blood bypasses the liver and is carried directly to the inferior vena cava by way of the ductus venosus [25]. The remaining umbilical vein enters the liver, where some of it joins the portal vein. The ductus venosus typically closes by the first week of life and is then known as the ligamentum venosum.
Liver function is not fully mature until about two years postnatally. Initially, its primary role is to regulate glucose and fatty acid metabolism to maintain a supply of energy for neonates that may not be getting adequate enteral nutrition in the first days of life. It is also responsible for the production of clotting factors and serum proteins, bile synthesis, and the biotransformation of medications and other xenobiotics, as well as the endogenous metabolic byproducts.
Maturation of the many liver functions occurs at differential rates. Albumin synthesis is at adult levels at birth in term infants. The coagulation factors (all are synthesized in the liver except for factor VIII) are initially low at birth but reach adult levels by 2–3 days of life. The expression of the hepatic enzyme uridine diphosphate glucuronyl transferase is poor in the fetus, but by age 2–3 weeks postnatally it reaches adult levels. This enzyme is needed to conjugate bilirubin in order to facilitate biliary excretion into the enteric system. Some of this conjugated bilirubin is then unconjugated by intestinal B glucuronidase and then reabsorbed into the body by way of the enterohepatic circulation. It is very common for term infants to have neonatal jaundice in the first few days to weeks of life because of increased erythrocyte breakdown, deficiencies of their ability to conjugate free bilirubin, and decreased amounts of enteric organisms available to break down unconjugated bilirubin for fecal excretion [26]. Clearance of medications depends on either a drug metabolism pathway or a hepatic transport mechanism. Metabolic pathways are usually divided into phase 1 reactions that involve oxidation, reduction, hydrolysis, cyclization, and decyclization reactions, and phase 2 reactions that involve the addition (conjugation) of polar groups to phase 1 metabolites [25]. Neonates are unable to metabolize medications as rapidly as adults because the cytochrome p450 system (which oxidizes medications) does not reach adult levels until one year of age [27]. For phase 2 metabolism, infants preferentially must use sulfation rather than glucuronidation for conjugation reactions [28]. Table 1.1 summarizes the maturation of liver functions.
Oxidative enzyme activity | Glucuronide conjugation | Sulfate conjugation | Effect on drug metabolism | |
---|---|---|---|---|
Premature neonates | Decreased oxidative enzyme activity (20–70 percent of adult values for cytochrome p450 activity) | Decreased glucuronide conjugation | Slightly decreased sulfate conjugation | Decreased |
Neonates | Same as premature infants | Same as premature infants | Slightly decreased from adults | Decreased |
Infants | Cytochrome p450 reaches adult activity by 6–12 months of age | Reaches adult levels by 24 months of age | Reaches adult levels in early infancy | Decreased |
Renal
The kidney matures over the first two years of life. Nephrogenesis is completed by 36 weeks postconception but the nephrons are immature at birth. During fetal development the primary role of the kidney is to maintain amniotic fluid levels and renal blood flow is a very small percentage of the fetal cardiac output. For the first week of life, renal blood flow is only 10 percent of cardiac output and does not reach the adult level of 25 percent until 24 months of life [29]. The glomerular filtration rate (GFR) is also diminished for the first two years of life. At birth it is about 40 ml kg–1 1.73 m–2 and reaches 66 ml kg–1 1.73 m–2 at two weeks of age, which is about one-half of the normal adult GFR [30]. The nephrons for the first few days of life are very immature and allow for some resorption of creatinine, which makes creatinine clearance a poor estimate of GFR during this period. These leaking nephrons rapidly mature and then creatinine levels drop.
One of the main functions of the kidney is to maintain fluid and electrolyte balance. At birth the extracellular volume is approximately 40 percent by body weight; this decreases to about 30 percent at six months of age and 20 percent by one year of age. Term infants generally do not have difficulty maintaining sodium balance, but excessive administration of sodium can overwhelm their kidneys, leading to peripheral edema. Likewise, the term kidney is able to maintain potassium, calcium, and phosphorus balance. Acid–base status is accomplished through ventilation, buffering capacity of serum proteins, bicarbonate-carbonic acids, hemoglobin–oxyhemoglobin and phosphorus, and the renal system. These mechanisms are all mature enough within three days of birth to handle nonrespiratory-induced acid loads.
The kidney also produces renin, which triggers the formation of angiotensin, which is converted to angiotensin II by angiotensin-converting enzyme. This enzyme increases the peripheral vascular resistance and cardiac contractility, leading to an increase in systemic blood pressure. The excretion of renin increases after birth and is higher in infants than older children. The renin angiotensin system, other humoral agents, and an increase in sympathetic activity at birth are responsible for the increase in blood pressure seen over the first weeks of life.
Neurologic
The neonatal and infancy period represents a time of dramatic brain growth, with the brain size as a neonate being 36 percent of the size of an adult brain and growing to 70 percent of its adult size by one year of age and 80 percent by two years of age [31]. During late gestation, brain development includes gray and white matter myelination, synaptogenesis, pruning, and synaptic modification. These processes continue into early infancy. Although all the cortical layers of cells are present at term and the primary cortical areas such as motor, somatosensory, visual, and auditory cortices are morphologically identifiable, the association cortices are less delineated. There is pronounced gray matter growth in the parietal and occipital areas in the first month of life. The newborn brain still exhibits a small degree of cortical neurogenesis and neuronal migration, but the dramatic brain growth is mainly due to the growth of glia and subsequent myelination during infancy. Abnormal brain growth in infancy is often a harbinger of poor neurocognitive outcomes. Microcephaly can be a result of malnutrition, and macrocephaly has been associated with autism.
MRI and autopsy studies of infants in the first year of life reveal a robust expansion of gray matter by way of elaboration of dendrites, spines, and synapses. Conversely, this is also a time of increased apoptosis or pruning. The pruning process time course begins with the primary cortices followed by the association cortices, and lastly in late childhood by pruning in the frontal lobes.
Along with synaptic pruning, there is remodeling of existing synapses and fine-tuning of neural circuits. Synaptic remodeling is dependent on the strengthening of certain synaptic pathways and weakening of others. Some of these are time-dependent during infantile maturation, such as the development of binocular vision. Lack of input from an eye with a congenital cataract will lead to potentiation of synapses from the functioning retina and inhibition of synapses from the poorly stimulated retina. This in combination with pruning can lead to permanent visual impairment in infants who get delayed treatment for congenital cataracts.
There is a dramatic increase in the volume of glial cells postnatally, which leads to an increase in myelination during the first year of life. Myelination proceeds in a differential pattern starting with the sensory pathways, then motor and finally association pathways. It also proceeds subcortically before cortically in any given neural pathway. At birth in full-term infants, there are functional networks that include the visual, sensorimotor, and auditory processing networks.
The effects of harmful toxins, illness, and environmental deprivation may be greater in very young infants whose brains are growing rapidly in a time-dependent fashion. The long-term effects of general anesthetics on the neonatal brain have not been adequately characterized yet.
Dermatologic
The term newborn has a dermis that is 40–60 percent the depth of adult dermis, and a larger surface area to body weight ratio than adults [32]. This means that the infant is more likely to lose heat and body fluids as well as absorb any materials placed on the skin compared to an adult. The pH of adult skin is acidotic at 4.7, which is protective by decreasing the colonization of pathogenic bacteria. At term birth, the pH is 6.0 but decreases to less than 5.0 by four days of age. The skin colonization of neonates resembles adult flora after the first few weeks of life. There are differences between the skin biome of infants delivered vaginally compared with caesarian section, with 60–82 percent of neonatal methicillin-resistant staphylococcus infections occurring in surgically delivered babies [33]. The vernix caseosa is a sebaceous material made from sebum, desquamated skin, water, and lanugo that is present in term infants, which enhances the skin barrier to infection, heat, and fluid loss.
The best type of disinfective skin preparation for surgery has not been determined. Many studies have shown that chlorhexidine in term infants can be safely used perioperatively and decreases surgical site infections [34–36]. There is evidence that chlorhexidine absorption does occur and increases after repeated exposures. Povidine iodine is an excellent bacteriocidal agent but causes thyroid suppression secondary to iodine absorption [37]. This effect is greater in premature infants but even in infants less than three months of age, increased levels of serum iodine have been found after skin exposure. Skin rashes and burns have been seen after surgical preparations in term infants exposed to isopropyl alcohol, chlorhexidine, and povidine iodine.
There are several very common rashes seen in term babies. Erythema toxicum neonatorum affects almost 50 percent of term newborns and is heralded by an erythematous macular papular rash with pustules. It usually occurs in the first week of life and lasts a few days. Milia are pinpoint pearly white bumps seen most often on the nose, mouth, or palate which are also seen in 50 percent of neonates and generally resolve by the first month of life. Neonates often get miliaria or heat rash from overbundling. It is caused by occlusion of the sweat ducts and features 1–3 mm vesicles or papules. Treatment is to limit humid or hot environments. Neonates are also susceptible to neonatal acne [38]. This is also self-limited and resolves as circulating maternal hormones diminish.
Endocrine
There are many endocrine changes that occur perinatally. Normally neonatal blood glucose levels drop in the first few hours of birth, sometimes to levels below 30 mg dl–1. A compensatory stress response involving the secretion of epinephrine and cortisol increases the plasma glucose to levels above 40 mg dl–1. There are no universally agreed guidelines as to the definition of hypoglycemia in term infants, but many neonatologists consider a plasma glucose of <40 mg dl–1 in the first three hours of life, <45 mg dl–1 in the first day of life, and <60 mg dl–1 after three days of life to be abnormal [39]. Infants who are born of diabetic mothers, large for gestational age, or have suffered severe perinatal stress or asphyxia may have transient hyperinsulinemia. Glucose requirements in excess of 8 mg kg–1 min–1 in order to maintain normoglycemia suggests a hyperinsulinemic state. Because insulin inhibits ketone body formation, decreases glycogenolysis and gluconeogenesis, and increases peripheral glucose uptake by cells, the brain is very vulnerable to damage such as permanent injury and seizures during hyperinsulinemia.
Thyroid homeostasis is necessary for brain development and growth, cardiac function, and energy balance. Prior to birth, the neonate is primarily dependent on maternal T3 and T4, but postnatally there is a dramatic increase (up to a 50-fold increase) in thyroid stimulating hormone (TSH) over the first 24 hours. It is important to recognize that a TSH in the first day of life in the 60–80 mU/L range is not indicative of thyroid disease [40]. One of the most common causes of abnormally low thyroid hormone levels in term infants is nonthyroidal critical illnesses, but there is no evidence that treating low levels benefits these patients [41,42]. Other causes include excessive iodine intake such as may be seen in iodinated contrast dyes or surgical preparations, and inadequate iodine intake. Congenital hypothyroidism is rare and is usually tested for at birth in the United States. Transient hyperthyroidism occasionally occurs in infants exposed to maternal hyperthyroidism.
At birth the adrenal system is mature, with adrenocorticotropic hormone (ACTH) stimulating the adrenal gland to secrete cortisol. Primary adrenal insufficiency is most often seen in infants that lack the enzyme 21 hydroxylase, which is necessary to synthesize corticosteroids and aldosterone. The symptoms include life-threatening salt wasting, hypotension, and poor growth. Affected infants also have very high levels of 17 hydroxyprogesterone and other adrenal androgens, leading to viralized infants. Female infants will have abnormal external genitalia but male infants may appear normal leading to a delayed diagnosis. Secondary adrenal insufficiency is common in extremely stressed term infants or in infants that have been treated with exogenous steroids. The mortality for infants with known adrenal insufficiency in the setting of sepsis is as high as 86 percent [43]. Treatment with glucocorticoids in infants with known adrenal insufficiency will improve blood pressure. Aldosterone is stimulated by the renin angiotensin system and thus is unaffected by low ACTH levels.
Serum calcium levels are regulated by parathyroid hormone release and special calcium-sensing receptors within the parathyroid glands. Parathyroid hormone (PTH) stimulates bone resorption to mobilize skeletal calcium and conversion of inactive vitamin D to active vitamin D to increase dietary absorption of calcium and phosphate. Transient hypoparathyroidism is seen in infants who are hypomagnesemic and infants born of mothers with diabetes mellitus and hyperparathyroidism [39]. Abnormal thyroid gland development is seen in DiGeorge’s syndrome, which can lead to hypocalcemia. Hypercalcemia of the neonate is most commonly iatrogenically caused, but is also seen in patients with Williams syndrome. In this condition, the hypercalcemia usually resolves by one year of age. Infants with Williams syndrome can present with pulmonary artery stenosis and supravalvular stenosis with associated coronary artery abnormalities. Sudden death from this syndrome is rare during infancy but has occurred.
References
Background on the Preterm Newborn
The preterm newborn is defined as a baby born from 22 to 36 weeks postconceptual age (PCA) [1]. More specifically, preterm is defined by the Center for Disease Control as less than 37 completed weeks of gestation, with early preterm being less than 34 weeks of gestation and late preterm being 34–36 weeks [2]. There were 3.95 million live births in the United States in 2011, with the preterm birthrate declining for the fifth year in a row, with a rate of 11.72 percent of all births being younger than 36 weeks, down from 11.99 percent in 2010 [2]. Overall, 0.7 percent of births in 2011 were babies younger than 28 weeks PCA (Figure 2.1) [3].
Figure 2.1 Total, early, and late preterm birth rates in the United States.
Categorization of birth age allows for stratification of risk. Data show that survival is least favorable in the smallest and youngest patients. Recently, with greatly improved accuracy in gestational age (GA) measurements, it has become clear that perinatal complications most closely follow GA, not birth weight. In extremely low gestational age newborns (ELGAN) born at 22–28 weeks PCA, there is improved survival with longer gestation as there is about a 6 percent survival to discharge in a 22-week GA newborn and 92 percent for a 28-week GA newborn [1,4]. The survival in very low birth weight patients has increased since the 1980s due to advances in prenatal, obstetric, and neonatal care, and we now see a majority of infants born at 24 weeks GA survive to leave the hospital (Figure 2.2) [4].
Medically intervening in newborns born at 22–24 weeks’ gestation is a controversial area of obstetrics and neonatology and it is not possible to standardize the data available [4]. We see that every week in utero confers better survival as the risk of death is 2.5 times higher in infants born from 24 to 27 weeks compared to those who are born at 28 weeks and more than three times higher for the 22-week newborns [4]. However, morbidity in the survivors is high in the 22–28 week PCA group and one report showed that 93 percent of these patients will have respiratory distress, 46 percent will have patent ductus arteriosis (PDA), 16 percent will have severe intraventricular hemorrhage (IVH), 11 percent will have necrotizing enterocolitis (NEC), and 36 percent will have late sepsis [4]. The most common issues for the smallest of patients are bronchopulmonary dysplasia (BPD) and infections [4]. All of these issues in and of themselves may require surgical attention or add to the anesthetic risk if the patient is taken to surgery. Other common problems that are seen in or result from preterm birth include: retinopathy of prematurity (ROP), hypoglycemia, and neurodevelopmental delay [1,4].
Pediatric anesthesiologists must be vigilant and thoughtful in the provision and planning of care for all of their patients. Preterm newborns who present for surgery are uniquely challenging due to the immaturity of all the organ systems, the complexity of their ongoing medical care, and the often emergent reasons for any surgical procedures. It is unlikely that the surgical issues these patients deal with today will change drastically over the coming years as there seems to be a current plateau in survival [4]. That being said, these patients are usually not cared for on a daily basis by pediatric anesthesiologists and this fact contributes to their challenging care.
Neurologic Issues
Over the past few decades, anesthetic care for preterm newborns has improved as research has allowed us to understand their complex physiology. We have come to learn that fetuses at 20 weeks’ gestation have the neural substrate to transmit impulses due to noxious stimuli. All newborns the pediatric anesthesiologist deals with will require either pain medications or anesthetics to minimize the detrimental stress responses related to surgical procedures [5]. Currently, there are concerns about the long-term neurologic sequelae of exposing the developing brain to anesthetics. This issue is far from resolved and clinical studies are underway that may add to our limited understanding of the effects of anesthetics on the developing CNS. Through vigilant observation and studies, practices are changing to optimize care. There has been recent change in NICU practice to avoid long-term midazolam administration to preterm newborns since this practice has led to the development of choreiform movements in these patients [1,5].
Preterm newborns are at risk for intraventricular hemorrhage (IVH), bleeding from the highly vascular periventricular germial matrix. IVH is multifactorial in nature, and almost 12 000 premature infants develop it every year [4,6]. Preterm newborns are at risk for neuronal injury, whether or not they require surgery, but changes in cerebral blood flow, possibly low or high blood pressure, swings in serum osmolalities, hypercarbia, thrombocytopenia, the presence of PDA, and restlessness are factors that may contribute to IVH [5,6]. The preterm brain lacks the ability to autoregulate blood flow on a consistent basis and this is a prevailing belief that predisposes them to neuronal injury [6]. Lower gestational age, lower birth weight, sicker, ventilated, and clinically unstable infants have been shown to autoregulate cerebral blood flow less well, and mortality is higher in these infants even without IVH [6]. Other types of brain injury are also commonly seen in preterm newborns; the “encephalopathy of prematurity” is a complex amalgam of destructive brain disease as well as maturational and growth dyscrasias affecting this population [7]. One of the most common manifestations is periventricular leukomalacia or disease of cerebral white matter, and its sequelae can be implicated in long-term cognitive and behavioral deficits found in up to 50 percent of formerly preterm newborns [7]. Neuronal damage can be caused by maternal infection, but also can be caused postnatally by sepsis, excitotoxicity, and inability to attenuate free radicals [7]. Also, ventilatory strategies may contribute to or protect infants from neuronal damage. Using pancuronium in intubated newborns to reduce asynchrony from the ventilator has been shown to eliminate fluctuations in cerebral blood flow and decrease risk for IVH [6]. Synchronized intermittent mandatory ventilation and assist control also reduce the fluctuation of cerebral blood flow in infants [6].
Pulmonary Issues
Preterm newborns have a host of respiratory issues that the pediatric anesthesiologist has to deal with, due to maturation, neurologic, chemical, and structural factors.
Respiratory regulation in the preterm newborn can be drastically different from that of the term infant. Postconceptual age is more of a determinant for mature responses to hypoxemia than postnatal age [8]. Periodic breathing occurs in most preterm newborns during REM sleep. Respiration becomes more regular as the newborn matures [8]. The risk of apneic spells is up to 45 percent in infants younger than 48 weeks PCA and more common in younger children [5]. Apnea is defined as cessation of breathing for greater than 20 seconds or cessation of breathing accompanied with a heart rate below 100 bpm, cyanosis, or pallor [5,8]. Preterm newborns are at risk for cardiac arrest if the apnea is prolonged, thus they require close monitoring [8]. Sepsis, intracranial hemorrhage, metabolic acidosis, lack of normothermia, vagal reflexes and anesthetics all contribute to apnea [9]. Preterm newborns may also develop apnea after receiving opiates until around 55 weeks PCA. An additional risk factor for postoperative apnea is a hematocrit of less than 30 percent [1]. An oxygen-enriched environment will promote regular respirations in the preterm newborn and a hypoxic environment will increase apneic spells and respiratory variations [8], which is important with regards to post-anesthesia considerations for the preterm newborn. With vigilance and close monitoring, apnea may be treated with stimulation, bag-mask ventilation, and addition of caffeine or assisted ventilation with continuous positive airway pressure (CPAP) or endotracheal intubation [5]. Obstructive apnea may also occur postoperatively in the preterm newborn due to excessive relaxation [8]. For elective surgeries of the preterm newborn, it is recommended to wait until the newborn reaches almost 60 weeks PCA to decrease this risk of postoperative apnea [5].
Chemical factors also play a critical role in the preterm newborn’s respiratory state. Surfactant, discovered by Dr. Mary Ellen Avery, is a substance that allows small alveoli to remain open in the presence of increased surface tension. Mature surfactant prevents the collapse of smaller alveoli into larger alveoli, thus maintaining functional residual capacity (FRC). Surfactant is made by pneumocytes after 24 weeks’ gestation. The composition changes during gestation and it is not until about 36 weeks’ gestation that there is sufficient mature, functional surfactant to prevent neonatal respiratory distress syndrome (RDS) [5,10]. Respiratory distress syndrome is due to a lack of surfactant, leading to decreased functional capacity [5]. Ninety percent of babies born at 26 weeks will experience RDS [5]. When requiring intubation and ventilation for surgery, these patients experience ventilation perfusion mismatch and may require additional support of PEEP [5]. Ventilation can be difficult and barotrauma and oxygen toxicity are risks of artificial ventilation in this age group; thus it is recommended to use the minimal concentration of oxygen necessary as well as to limit the peak inspiratory pressures used [5]. Because preterm newborns have decreased endogenous surfactant and will often develop respiratory distress, they may be treated with intratracheal surfactant (“surfed”) in the NICU. Intratracheal surfactant has been shown to reduce air leaks, improve survival, and reduce the severity of chronic lung disease [9]. Despite the advantages of surfactant administration, the incidence of BPD in babies treated with surfactant has not been decreased and the use of surfactant in babies born at 28 weeks has decreased in recent years. Bronchopulmonary dysplasia is the requirement of supplemental oxygen at 28 days of life with a chest radiography demonstrating air trapping, atelectasis, and lung field opacification [9]. Assessment of the patient’s respiratory status is important to drive decision-making and treatment plans about the use of surfactant. For example, if the patient is spontaneously breathing, requiring less than 0.4 FiO2, then using a trial of nasal CPAP may be practiced in favor of intubating and instilling surfactant (Figure 2.3) [4,9].
Structural lung difference in the preterm newborn also contributes to their more delicate respiratory status. Preterm newborns have reduced lung volumes compared to term newborns. There is a four-fold increase in lung volume and alveolar surface area from 25 weeks to term. Preterm newborns also have a very compliant chest wall, which predisposes them to injury both via volutrauma (pneumothorax) and barotrauma (BPD) when positive pressure ventilation is used [1]. If intubated, NICU strategies are currently incorporating permissive hypercapnea, especially if PIPs are higher than 14–18 cmH2O, and oxygenation goals are aiming for saturations in the upper eighties to decrease damage to the lungs [1]. A parameter of preterm newborn assisted ventilation is to prevent hypoxia, while at the same time avoiding hyperoxia, and it can be a delicate balance. A low range SpO2 of 85–89 percent decreased retinopathy of prematurity, but increased mortality, in very low birth weight (VLBW) newborns on supplemental oxygen [9]. Hyperoxia plays a role in decreasing ciliary movement and adversely influencing bronchopulmonary development. The inspired oxygen concentration of oxygen seems to be the main factor, rather than the arterial concentration [9]. PEEP is often utilized in intubated preterm newborns, at around 5 cmH2O, with the goal of keeping distal airways open to reduce shear stress from when they repeatedly close and reopen, improving oxygenation, and allowing down-titration of inspired oxygen [1,9]. Goal tidal volumes are often in the 6 ml kg–1 range [1].
One must also consider the small size of the airway when choosing endotracheal tubes. The consideration of cuffed versus uncuffed tubes for risk of subglottic stenosis must be addressed. Also, if a patient is particularly small or requires sophisticated ventilation such as high-flow oscillatory ventilation, it may be beneficial to continue the NICU ventilator in the operating room in order to maintain clinical stability
Cardiac Issues
The preterm newborn is at high risk for cardiovascular compromise when exposed to anesthetic agents and surgery. The physiology of the immature heart consists of poor diastolic function, fixed stroke volumes, and poor autoregulation of the systemic vasculature [5]. Patients administered potent inhalational anesthetics have impaired baroreceptor reflexes and compensate poorly, if at all, for blood loss and hypovolemia [5]. The pediatric anesthesiologist also must have an awareness of congenital anomalies of premature infants as they are 2.4 times more likely to have cardiovascular malformations than term newborns [11]. Preterm newborns are more likely than full-term babies to be born with pulmonary atresia with ventricular septal defect, complete atrioventricular septal defect, coarctation of the aorta, Tetralogy of Fallot, and pulmonary valve stenosis [11]. Also, a majority of preterm infants have a patent ductus arteriosus (PDA) that may require medical or surgical intervention [1,5]. In the term newborn, there is a rapid decrease in pulmonary vascular resistance due to a chemical response to increased pO2, as well as a reduced pCO2, and intrapulmonary cardiac and pulmonary pressure changes lead to a quick closure of the patent foramen ovale (PFO) and a PDA [8]. In preterm newborns there is a delay in the response to the increase of arterial oxygenation with extrauterine respirations. When preterm newborns become hypoxic, hypercarbic, or acidotic, they may revert back to a fetal circulation due to a relatively quick increase in pulmonary vascular resistance. This may result in right-to-left shunting via the PFO or opening of the PDA [8]. Monitoring of preductal (right upper extremity) and postductal (lower extremity) saturations is a common intraoperative practice of anesthesiologists that care for the preterm newborn. An open PDA with left-to-right shunting contributes to morbidity in the form of IVH, pulmonary edema and heart failure, NEC, renal insufficiency, or hemorrhage [1].
“Normal” blood pressures are difficult to define in the preterm newborns, but one may review recent blood pressures obtained to have a guide of what “normal” is for a particular patient. Intraoperative blood pressure measurement must use the same size non-invasive blood pressure (NIBP) cuff as was used prior to the trip to the operating room. Hypotension is found in up to 45 percent of premature infants, with hypertension being less common [6]. A common practice among pediatric anesthesiologists caring for patients in this group is to treat the blood pressure if the mean is lower than the GA plus 5 mmHg [5]. Blood pressure treatment can be with colloid, crystalloid, or vasopressors [5]. It is important to note that stroke volume is 1 ml kg–1 and left ventricular end-diastolic volume is 2 ml kg–1. Calcium supplementation may be helpful in augmenting blood pressure because preterm newborns have poor calcium reserves and their myocardial function is driven by extracellular calcium [1].
Gastrointestinal Issues
The immature gastrointestinal tract of the preterm newborn requires consideration by the anesthesiologist. It is common for these patients to have reflux due to poor esophageal sphincter tone and impaired peristalsis [5]. Gastrointestinal reflux can contribute to aspiration, cough, laryngospasm, and other respiratory compromise that contribute to morbidity related to anesthetics [5]. Necrotizing enterocolitis can develop in the preterm newborn for many reasons and must be considered in these patients. Etiologic factors for the development of NEC are impaired intestinal blood flow, early and hyperosmolar feeds, and prematurity. Bowel perforation associated with NEC can lead to sepsis [5]. If the NEC does not respond to medical treatment, the patient may require an emergent exploratory laparotomy.
Hematology Issues
Preterm newborns may present to the operating room relatively anemic and require transfusion after surgical blood loss. Anemia of prematurity is multifactorial and stems from delivery prior to placental transport of iron, incomplete fetal erythropoesis, recurrent phlebotomy of the hospitalized infant, low erythropoietin production, and rapid growth [9]. Preterm newborns, especially extremely low birth weight newborns, are likely to have received a transfusion if they have cardiopulmonary issues as the increased oxygen-carrying capacity of red blood cells will aid in patients with cardiorespiratory distress, though possibly increasing the risk of NEC [9]. The absolute blood volume of the smallest of patients is in the 100 ml kg–1 range [5]. A few milliliters of blood loss during surgery may be a significant percent of total blood volume. The newborn born at less than 28 weeks will have hemoglobin levels of 13–15 g dl–1, with 75 percent of it being fetal hemoglobin, which does not release oxygen as readily as adult hemoglobin [5]. Preterm newborns also have immature coagulation profiles with low platelet counts and vitamin K deficiency. All newborns should receive vitamin K if going for surgery [5]. Transfusion in these patients must be done after warming the blood, done in small volumes, and given slowly, with consideration of washing the blood to decrease the potassium load delivered over a short amount of time. A 10 ml syringe of packed red blood cells pushed quickly in a 1 kg weight baby via an IV increases the blood volume by 10 percent, which is analogous to pushing two units of packed red blood cells into an adult IV over less than one minute [1,5].
Hepatic Issues
The immature liver of preterm newborns is not able to metabolize medications as well as a mature liver, does not synthesize certain proteins well, affecting drug metabolism, and does not have glycogen stores. Preterm newborns require the same glucose levels as term newborns, but because of their limited glycogen stores glucose must be supplemented when these babies are stressed or having feeds held in preparation for surgery [5]. Hypoglycemia is detrimental and may be difficult to recognize in these small patients, but hyperglycemia also carries an increased risk of intraventricular hemorrhage, dehydration, increased length of hospitalization, and death [5,9]. Infants most at risk for hyperglycemia, defined as >150 mg dl–1 of glucose, are preterm newborns <1000 g, those receiving glucose infusions of >6 mg kg–1 min–1, those with illness, and those on glucocorticoid therapies [9]. Treatment of hyperglycemia can include decreasing glucose infusion rates, early amino acid administration to increase insulin release, and possibly instituting insulin infusions. Insulin infusions should be used with extreme caution, given the small doses involved and the significant morbidity associated with hypoglycemia, which may be difficult to recognize in the anesthetized patient [9].
Renal Issues
The preterm newborn is at risk for hyponatremia since renal tubules do not have the ability to retain sodium until 32 weeks’ gestation, the response of the distal tubules to aldosterone is low until 34 weeks’ gestation and antidiuretic hormone levels are elevated [5]. Negative sodium balance is not uncommon in preterm infants of less than 35 weeks’ gestation and this negative balance may last for up to three weeks after birth [9]. Hypernatremia may occur if there are insensible fluid losses not adequately replaced [1]. Newborn acid–base status is often on the acidotic side and in the first day of life they may show combined metabolic and respiratory acidosis. The renal tubules are not able to reabsorb bicarbonate well and there is normally mild metabolic acidosis seen in these patients [5]. For the first month they may have metabolic acidosis due to a net acid input from metabolism, protein intake, and bone mineralization with a decreased capacity for acid excretion. This acidosis increases the risk of negative calcium balance and poor bone formation [9], which can impact cardiac function and fracture risk, respectively.
Skin/Temperature Maintenance Issues
The epidermis of preterm newborns is very thin and requires the utmost of care and consideration. Preterm infants who are hypothermic on admission to the NICU after birth are at high risk for mortality [9]. There is a great amount of heat loss due to the large surface area to body size ratio, lack of adipose tissue, and thin skin. Infants less than 2 kg in weight are particularly efficient in transferring heat from the internal to external gradient [8,9]. Hypothermia leads to a decreased respiratory response to hypoxia. Infants born between 32 and 37 weeks will have an increase in ventilatory rate in response to hypoxia in a warm environment; this response is not seen in a cool environment [8]. Temperature monitoring and maintenance are very important in the preterm newborn. In environments of high temperature or when the environmental temperature fluctuates, these infants are at risk for apnea [9]. Their thin skin is also easily bruised and/or abraded by tape and monitors. These injuries are similar to partial thickness burns.
Total body water in these patients is 80 percent of body weight, and this amount decreases as the infant matures. This amount of fluid paired with the thin skin leads to evaporative fluid losses 15 times that of a full-term newborn in the first days of life. Newborns younger than 32 weeks are at the highest risk because they have the thinnest epidermal layer, but the skin does thicken over weeks of extrauterine life [1,5]. Also, preterm newborns rely on non-shivering thermogenesis, which includes utilization of their richly vascular and innervated brown fat, which has mitochondrial oxidative metabolism, to produce heat [9].
Common Surgical Conditions of the Preterm Newborn
Common surgical conditions of preterm newborns include:
Cut-downs for IV access
Necrotizing enterocolitis exploratory laparotomy
Patent ductus arteriosis ligation
Inguinal Herniorrhaphy
The most common surgery performed in preterm newborns is inguinal herniorrhaphy. Thirty-eight percent of infants with a birth weight from 751 g to 1000 g will have an inguinal hernia, and since these hernias are larger than those seen in term newborns and infants, the risk for incarceration or strangulation is greater. The incidence of inguinal hernias is approximately 15 percent in infants born weighing 1001–1250 g [5]. Up to 49 percent of these babies are at risk for postoperative apnea [9].
Preparation for Surgery
The pediatric anesthesiologist must review the patient’s birth history, age at birth, current age in terms of PCA, and current weight. For all but the most minor procedures in relatively well preterm newborns, a complete blood count and coagulation profile should be obtained. Chest X-rays or echocardiograms should be reviewed to assess for significant cardiac or respiratory anomalies.
Guardians of preterm newborns presenting for surgery should be aware of the higher anesthetic risk. The anesthesiologist should review the possibility of difficulties in managing the airway, issues associated with intubation and having a breathing tube, the possibility of postoperative intubation, difficulties with vascular access, and the possibility of cardiorespiratory compromise and need for blood transfusion or blood products [5].
Premedication is not usually required as these small patients do not have perioperative anxiety or stranger awareness/anxiety. Atropine for prophylaxis against anesthesia-induced bradycardia is not as commonplace as in the past with current inhalational agents, but the correct dose of IV atropine should be immediately available [5].
The operating room thermostat should be kept very warm prior to arrival of the patient (27 °C/80 °F) and warm lights and hot air warming devices should be employed to maintain a neutral thermal environment for the patient [5]. Monitoring patient temperature must be done with care as esophageal or rectal probes may cause perforation even when placed gently [1,5]. Temperature should be maintained carefully so as to not cause hyperthermia [9]. Fluids and blood products should be warmed and given slowly.
Standard monitors should include pre- and postductal pulse oximeters. Noninvasive blood pressure cuffs should be appropriately sized as a large cuff may falsely read lower than the actual pressure, and placement should be careful as the bones are at risk for fracture [5]. The upper extremity blood pressure cuff may be a more reliable reading for mean arterial pressure, but may not be practical if lines are in place or if the extremity is part of the operative field. Invasive monitors are often extremely technically challenging and attempts to place them in very small patients may confer more harm than benefit, thus they may not be employed [1,5]. Some preterm newborns will present with umbilical lines in place and the arterial line can provide useful information or allow for easy lab draws; however, rapid flushing or drawing off of the line may cause morbidity and it should be used carefully [5]. The anesthesia machine used should be able to deliver reliably small volumes, PEEP, and limit pressure delivered to the patient to minimize barotrauma and optimize ventilation. If a patient is particularly small, a neonatal ventilator from the NICU may be required.
The patient may arrive already intubated, but the anesthesia team should be prepared if extubation occurs and have small face masks available, Miller 0 and 00 blades checked and ready to be used, a size 0 LMA available, and endotracheal tubes down to size 2.5 outer diameter with small stylets [1,5]. It is also important to have the proper size suction catheters available (a 5 French catheter for a 2.5 endotracheal tube, a 6–7 French catheter for a 3.0 endotracheal tube, and an 8 French catheter for a 3.5 endotracheal tube). The distance from vocal cords to carina in the trachea in preterm newborns may be less than 4 cm [5]; thus, care must be taken in tube placement and continuous assessment for extubation or mainstem intubation. A reference of tube length to guide for where to tape an oral endotracheal tube (OETT) at the gum of preterm newborns of various weights is to have the tip midtrachea; for newborns weighing 1, 2, 3, 4 kg the OETT should be taped at 7, 8, 9, 10 cm respectively [5]. A leak of the tube should be assessed. In order to minimize tracheal trauma and swelling, a leak should be audible between 10 and 30 cmH2O [5,9]. These small endotracheal tubes are easily bent and kinked, and a small amount of secretions may block the tube. The anesthesiologist must be cognizant of these common complications. If a patient comes to the operating room already intubated, it is important to note the position of the tube, and it is not recommended to change out the endotracheal tube unless there is dislodgement, excessive leak, or occlusion due to secretions [9].
Inductions may be safely achieved with fentanyl and a neuromuscular blocking agent [1]. Propofol has been used to provide good intubating conditions in preterm neonates requiring intubation for surfactant administration, but it has been found to cause prolonged hypotension in this population and should be used with caution [12]. Preterm newborns born at 29–32 weeks who received 1 mg kg–1 of propofol for intubation had a decrease in mean arterial pressure from 38 mmHg to 24 mmHg, ranging from 29–42 mmHg to 22–40 mmHg, respectively [12].
Maintenance of anesthesia should be done with moderate amounts of inhalational agents, such as sevoflurane, isoflurane, and desflurane. Halothane caused bradycardia and postoperative apnea in preterm newborns and is no longer is use [5]. Moderate amounts of inhalational agent should be dosed to avoid a decrease in systemic arterial pressures and to minimize increases in pulmonary vascular resistance [5]. Balanced anesthesia with fentanyl is commonly done as fentanyl provides cardiovascular stability while providing pain control, and is safe in patients at risk for, or with, pulmonary hypertension [5]. Many of the preterm newborns presenting for surgery will remain intubated and ventilated postoperatively, thus adequate dosing of fentanyl may be achieved. For the patients who will not remain intubated postoperatively, regional anesthesia with or without inhalational agents and acetaminophen will provide benefit [5]. A prospective study in 2006 showed that preterm newborns at less than 47 weeks PCA who presented for inguinal herniorrhaphy did not have postoperative apnea requiring airway intervention after an anesthetic with controlled ventilation with sevoflurane or desflurane, rectal acetaminophen 20 mg kg–1 and a caudal block with 0.25 percent bupivacaine 1 ml kg–1 [13]. Postoperative analgesia should be achieved with opiates only if the infant can be closely monitored, preferably in an ICU setting. Acetaminophen is the sole analgesic deemed safe for use in preterm newborns [5], as NSAIDs are not recommended for those under six months of age. Rectal acetaminophen is suggested for postoperative pain control after abdominal surgery in neonates, with a dose of 15 mg kg–1 around the clock every 12 hours for newborns born at <32 weeks’ gestation for four doses, or every eight hours for those patients born >32 weeks to 40 for six doses [9].
Once intubated, preterm neonates should not be exposed to high levels of oxygen. Oxygen toxicity to the lungs and developing retina is a concern in preterm neonates as high levels of oxygen administration contributes to morbidity and mortality [5,9]. It is recommended that after intubation is achieved, maintenance of inspired oxygen should be titrated to achieve oxygen saturations of 88–95 percent [5,9].
Regional anesthesia is safe in preterm neonates. Preterm neonates have a larger volume of cerebrospinal fluid compared to older children and they have an increased surface area around nerve roots, so medication dosing is higher and duration of block is shorter [5]. Blood pressure is well preserved when regional anesthesia is used, owing to the low vasomotor tone of preterm newborns and decreased vagal activity [5]. It is possible to place a spinal without IV access then secure lower extremity IV access once the spinal sets up. If a regional anesthetic is chosen for the preterm neonate, it should be noted that the procedure is technically more difficult than in term babies. One in seven attempts at spinal fails to find the intrathecal space, and one in three of these babies require additional anesthetic in addition to the spinal for adequate surgical conditions [5].
Intravenous fluids should be warm, free of air, and limited in volume delivered. Due to a high risk of hypoglycemia, dextrose-containing fluid given at maintenance should be part of the anesthesia care. This may mean glucose at a rate of 8–10 mg kg–1 min–1 or 10 percent dextrose at 110–120 ml kg–1 day–1 [1].
References
The Lion incubator was invented in 1891, but its approval for use in hospitals lagged behind. The first public use of an incubator for the care of an infant occurred in a side show of the Dreamland amusement park on Coney Island, NY and warmed a set of triplets born early to the owners of the show. Herein lies the birth of the specialized care of the premature infant in a world of modern medicine and technology [1]. A series of advancements, including portable incubators, ventilators, and antibiotics eventually led to the creation of the first official neonatal intensive care unit (NICU) at Vanderbilt University in 1961, when a ventilator was used (off-label) for the first time to help a struggling neonate.
While neonatology and pediatric anesthesia may not intuitively seem the closest of medical cousins, their developments have both relied heavily on technological advancements in order to care for ever sicker and more precarious neonates. The care of premature infants has continually fallen squarely into the hands of these two divisions, and an understanding of one another is paramount to the optimal care of patients in this unique population. The collaboration between the two groups is most notably recognized in the personage of Virginia Apgar (Figure 3.1), an anesthesiologist who developed the APGAR scoring system in 1952, to objectively assess the overall status of a newborn during the first moments of transition from fetal life [2].
Figure 3.1 Virginia Apgar, MD.
Population of the NICU
Patients
The National Perinatal Information System collects data on admissions to special care nurseries. “Special care” nurseries (SCNs) are defined as either NICUs or neonatal intermediate care units (NINTs), and include data on children born at hospitals with these units on site as well as children transported into these units. These data are prepared by the March of Dimes into a report titled “Special Care Nursery Admissions.” The most salient statistics include the following regarding infants born during the study period (July 1, 2009 to June 30, 2010):
14.4 percent of all infants were admitted to an SCN;
49.1 percent of admissions were preterm (defined as <37 weeks gestational age [GA]);
70 percent of these premature infants were admitted to the NICU, not NINT;
23.6 percent of infants admitted to the SCN had a primary diagnosis of “prematurity”;
11.9 percent of infants admitted to the SCN had a diagnosis of “respiratory distress syndrome”;
90 percent of infants born less than 34 weeks GA were admitted to an SCN [3].
Providers
As in other intensive care environments, the providers in the NICU are highly trained and varied in skillsets. The physician specialist associated with the NICU is the neonatologist (a pediatrician with subspecialty training in neonatology), though many NINTs are immediately staffed by pediatricians with neonatologist supervision. Other direct providers include neonatal nurse practitioners as well as neonatal clinical nurse specialists. In addition to these, and depending on the hospital environment, there may also be neonatology fellows and pediatric residents caring for the neonates. The success of an NICU depends on the collaboration of multiple providers working together as a team. Members of the team often include respiratory therapists, physical therapists, occupational therapists, speech therapists, registered dietitians, lactation consultants, pharmacists, and social workers.
Common Medical Issues in the NICU by Systems
The NICU population is unique in the medical system, and their problems and diagnoses are equally unusual. While other chapters in this book will look more exhaustively at each system described below, it is prudent in a discussion of the NICU to address the major conditions, abnormalities, and diagnoses found in the average NICU. We will address these conditions by systems.
Neurologic
Neurodevelopment is a process that is ongoing throughout childhood and adolescence, but it is particularly dynamic in the neonate, and especially so in the preterm neonate. A more detailed review of neonatal response to pain, development, and drug toxicity can be found in other chapters of this book. Below, we briefly discuss the major neurologic conditions often uniquely found in the neonatal population: intraventricular hemorrhage (IVH), hypoxic-ischemic encephalopathy (HIE), retinopathy of prematurity (ROP), and the neonatal abstinence syndrome (NAS).
Intraventricular Hemorrhage
The neonatal brain is a rapidly developing organ, with neuronal migration originating in the highly vascularized germinal matrix, which is a particularly delicate region, exquisitely sensitive to physiologic perturbations. With such changes, bleeding can develop within the germinal matrix; staging of IVH is based on the degree of blood spread into the periventricular parenchyma and adjacent lateral ventricles. Smaller infants are more susceptible to hemorrhage, especially within the first few days of life and since the presentation is often subtle, screening head ultrasounds have become routine for low birth weight neonates in most NICUs [4].
Hypoxic-Ischemic Encephalopathy
While HIE remains a relatively rare occurrence in neonates (1–3 per 1000 live full-term births), the morbidity and mortality remains high. Fifteen to twenty percent of infants with HIE will die during the postnatal course and another 25 percent will develop significant and permanent neurologic sequelae, such as cerebral palsy, neurodevelopment impairment, and epilepsy. Currently there is much research into neuroprotective strategies that can be employed following a hypoxic insult. The only proven strategy is that of hypothermia (cooling the infant by 3–5 °C), although many other strategies (e.g., free radical scavengers and erythropoetin) are being investigated [5].
Retinopathy of Prematurity
Premature infants are born with incompletely vascularized retinas. When subsequent vascularization does not proceed normally, ROP develops, which if left untreated could lead to retinal detachment and blindness. ROP generally occurs in two phases, with retardation in vessel growth occurring first at around 30–32 weeks post-GA and then a proliferative phase occurring at approximately 32–34 weeks post-GA. The most recognized risk factors are prematurity, low birth weight, and hyperoxygenation (including the use of unnecessary supplemental oxygen in the operating room), although infants exposed to very little supplemental oxygen have developed ROP. Improved screening, early diagnosis, and treatment (with bevacizumab [an angiogenesis inhibitor] or LASER treatment by an ophthalmologist) have improved the overall morbidity, despite the fact that the overall incidence of ROP has not declined [6].
Neonatal Abstinence Syndrome
As the number of people dependent on opiates (both prescribed and illicit) and other psychotropic medications has increased in the last few decades, so has the incidence of intrauterine exposure and the number of infants born dependent on these same substances. The signs and symptoms displayed by neonates as they withdraw from these medications are collectively referred to as the “neonatal abstinence syndrome” or NAS. The timing of first symptoms is dependent on the type and dosage of the exposed medication, with heroin and oxycodone withdrawal occurring rapidly and methadone and buprenorphine withdrawal sometimes not occurring for several days. Treatment generally consists of the controlled replacement and weaning of opiate (usually morphine or methadone), with or without adjuncts such as phenobarbital or clonidine, while carefully monitoring for signs of withdrawal. Various scoring systems and weaning strategies are used, and are quite unit-specific. Many units are utilizing nonpharmacologic management techniques such as occupational and massage therapy [7].
Cardiac
During fetal life, roughly 90 percent of combined ventricular output is directed toward the systemic circulation, with only 10 percent entering the pulmonary vasculature. This feat is accomplished mainly via two shunts: the intracardiac foramen ovale and the extracardiac ductus arteriosus; these two shunts close postnatally as the infant’s pulmonary vascular resistance falls and the infant transitions away from the fetal circulation. Excluding congenital heart disease (CHD) and the hypotension associated with sepsis, the bulk of cardiac pathology in the NICU concerns an abnormal transition to postnatal circulation.
Patent Ductus Arteriosus
The ductus arteriosus is a large conduit that links the fetal main pulmonary artery to the descending aorta, which usually closes early in postnatal life as oxygen tension increases and prostaglandin input decreases. Its closure is usually welcome, except in the case of so-called ductal-dependent cardiac lesions, but it often remains open in preterm neonates, leading to the unwanted shunting of blood. In fact, in infants born at <28 weeks GA, a majority (60–70 percent) will require either medical or surgical closure of a patent ductus arteriosus (PDA). There is considerable research into when a PDA is significant enough to warrant intervention, but two of the most important considerations are those of diastolic flow reversal and cerebral steal and pulmonary overcirculation. Once the decision has been made to intervene, a trial of medical closure is generally attempted, using cyclooxygenase inhibitors like indomethacin or ibuprofen. If medical treatment is unsuccessful or inappropriate, a surgical PDA ligation can be performed via a left thoracic approach [8].
Persistent Pulmonary Hypertension of the Newborn
As its name implies, persistent pulmonary hypertension of the newborn (PPHN) occurs when the normal transition from fetal to postnatal circulation does not occur. Normally, shortly after birth, the systemic vascular resistance increases (due largely to the clamping of the umbilical cord), the pulmonary vascular resistance drops precipitously (due to multiple factors), and the major fetal shunts (the ductus arteriosus and foramen ovale) experience a functional followed by anatomical closure. When this drop in pulmonary vascular resistance does not occur, the closure of the shunts cannot occur, and the neonate will remain in a fetal circulation characterized by a circuit in parallel instead of a mature circuit in series. In other words, if a neonate has PPHN, shunting and variable systemic oxygen saturation and perfusion can result. The causes of PPHN are varied, but are generally due to abnormal parenchyma with resultant vasoconstriction (e.g., meconium aspiration syndrome, respiratory distress syndrome, pneumonia), abnormally deficient vasculature (e.g., pulmonary stenosis, lung hypoplasia), or are idiopathic. Treatment focuses on overall physiologic stability and efforts to decrease pulmonary vascular resistance through interventions such as inhaled nitric oxide, phosphodiesterase inhibitors, sedation, and methods to optimize oxygenation. When conventional therapies are unsuccessful, extra corporeal life support (ECLS) is offered to a select population [9].
Congenital Heart Disease
An exhaustive discussion of CHD is beyond the scope of this section. We will instead focus on just those conditions that will present within the first few hours or days of life. The immediate neonatal period is marked by a transition from fetal circulation to adult circulation and can often bring to light major congenital cardiac lesions. Certain lesions, such as total anomalous pulmonary venous return and critical pulmonary stenosis, are not even apparent on fetal surveys due to the significantly decreased pulmonary blood flow in the fetus. Other lesions, such as hypoplastic left heart syndrome and transposition of the great arteries, require a functional ductus arteriosus in order to supply oxygenated blood to the systemic circulation. In ductal-dependent cardiac lesions (see Table 3.1), an infusion of prostaglandin E1 can maintain patency of the ductus arteriosus and even open a functionally closed ductus arteriosus if anatomical closure has not occurred. Airway control is an important consideration when using prostaglandins, given associated apnea.
Table 3.1 Ductal-dependent cardiac lesions
• Critical coarctation of the aorta (decreased systemic perfusion) |
• Interrupted aortic arch (decreased systemic perfusion) |
• Critical aortic stenosis (decreased systemic perfusion) |
• Hypoplastic left heart syndrome (decreased systemic perfusion) |
• Tricuspid atresia (decreased pulmonary perfusion) |
• Pulmonary atresia (decreased pulmonary perfusion) |
• Transposition of the great vessels (inability to deliver oxygenated blood to the systemic circulation) [10] |
Pulmonary
Lung immaturity is a predictable biochemical, physiologic, and anatomical phenomenon in premature infants, and this unique situation has led to the development of a different set of treatment and ventilation strategies than in adults and children.
The threshold of viability is accepted by many to occur at approximately 24 weeks GA, and this time corresponds with the embryological development of respiratory bronchioles and alveolar ducts and signifies the initiation of the ability to oxygenate and ventilate. At approximately 26 weeks GA, type II pneumocytes begin to populate the alveoli and begin the production of surfactant, which will ultimately prevent the collapse of alveoli with exhalation and dramatically improve pulmonary compliance [11].
Antenatal steroids are a mainstay in the treatment of women with preterm labor (less than 32–34 weeks GA) because of the known effect on fetal lung maturation and surfactant expression. The efficacy of antenatal steroids has been strongly validated, with multiple meta-analyses demonstrating a clear benefit [12].
One aspect of neonatal ventilation often not seen in older children is the relative immaturity of respiratory control that can lead to irregular breathing patterns and even life-threatening apnea. This is multifactorial, with causes ranging from altered response curves to hypoxia and hypercapnea, to immature brainstem and peripheral receptor networks. Furthermore, neonates do not seem to be able to handle an oxidant stress and are particularly vulnerable to oxygen toxicity. In fact, the 2010 American Heart Association Guidelines for neonatal resuscitation recommend initiating resuscitation for term infants with room air and blending in oxygen as needed to achieve an acceptable oxygen saturation [13].
Respiratory Distress Syndrome
Respiratory distress syndrome (RDS), previously known as hyaline membrane disease, is best understood as difficulty ventilating due to the lack of surfactant and subsequent decrease in lung compliance and propensity for alveolar collapse. It is most commonly observed in premature neonates due to their natural surfactant-deficient state, but can be observed in full-term neonates whose surfactant, while present, might be inactivated by a variety of causes. Treatment depends on the degree of distress and prematurity and can include exogenous surfactant administration, noninvasive ventilatory support, and invasive ventilation [14].
Chronic Lung Disease and Bronchopulmonary Dysplasia
Chronic lung disease (CLD) is a fairly generic term that refers to pulmonary disease that follows respiratory disorders of the neonate. Bronchopulmonary dysplasia (BPD) is a form of CLD with a specific definition: The need for supplemental oxygen at least 28 days after birth or the need for supplemental oxygen after 36 weeks corrected GA, depending on the definition being used. As one can imagine, this does not have a high predictive value for long-term respiratory dysfunction, as it does not take into account GA at birth or oxygen delivery method (e.g., nasal cannula versus endotracheal intubation). Bronchopulmonary dysplasia is a condition of prematurity, almost always occurring in infants born at less than 30 weeks GA and with low birth weight (under 1500 g). As improvements have been made to the treatment of RDS, fewer infants have developed BPD characterized by non-homogeneous parenchymal damage due to ventilator-associated trauma. Instead, as smaller and younger infants are now surviving, infants are now developing a more homogeneous BPD characterized by the reduced development of alveoli. Treatment is centered first on prevention. Frequent strategies employed may include the occasional use of diuretics to improve respiratory mechanics, optimizing nutrition, palivizumab for respiratory syncitial virus prophylaxis, short courses of steroids for exacerbations outside of the neonatal period, and inhaled bronchodilators for obstructive exacerbations [15,16].
Transient Tachypnea of the Newborn
As discussed in the section on cardiac physiology of the neonate, the newborn infant must undergo a dramatic transition in order to move from a liquid environment reliant on maternal ventilation and oxygenation to one where ventilation and oxygenation are paramount to survival. The fetal lung is filled with fetal lung fluid up to a volume that is close to what will ultimately become the functional residual capacity. A small portion of this fluid is expelled due to external forces during labor, but the majority is reabsorbed by the lung epithelium once labor commences and levels of epinephrine increase. This is likely the reason that infants born via cesarean section without labor have a significantly increased incidence of transient tachypnea of the newborn (TTN) compared to those born by cesarean section after the beginning of labor [17]. When this fluid is not reabsorbed quickly enough, retained lung fluid will cause the infant to display symptoms of tachypnea, grunting, retractions, and variable hypoxia. This is usually a self-limited condition that resolves within 2–3 days [18].
Ventilation Modes in the NICU
Given the physiologic challenge that incompletely developed neonatal lungs present, it is not surprising that neonatal ventilation strategies is an area of active research. There are multiple modes of noninvasive (i.e., without an endotracheal tube) as well as multiple modes of invasive ventilation utilizing endotracheal intubation. Because of the very small tidal volumes in the range of 4–6 ml kg–1 (sometimes less than 5 ml in extremely low birth weight infants), the ventilators and circuitry have to operate in a precise manner with highly sensitive flow and pressure sensors [19].
In recent years, controversy has emerged regarding the use of cuffed versus uncuffed endotracheal tubes. In the past, the thought was to not use cuffed tubes in children younger than eight years because of the natural narrowing at the level of the cricoid cartilage, and because of the potential morbidity of subglottic trauma being caused by the cuff. With newer, lower profiled cuffs, this does not seem to be as much of an issue, and many practitioners are moving toward cuffed endotracheal tubes for neonates. The advantages of a cuffed tube include a good fit with fewer intubations, as well as a good seal with a minimized leak. Despite this movement, subglottic edema and remodeling continues to be a major concern of neonatologists and many units continue to use uncuffed endotracheal tubes as their standard of care [20].
Noninvasive Modes
Nasal continuous positive airway pressure (NCPAP) has been well established as a method of noninvasive airway support in neonates, often used following extubation or in the attempt to prevent the need for endotracheal intubation. Recently, newer methods have developed using sensitive triggers and processors that can provide more advanced and timed support. Synchronized noninvasive positive pressure ventilation (SNIPPV) and non-synchronized noninvasive positive pressure ventilation (nsNIPPV) have also been studied. There is a Cochrane Database review showing a benefit of NIPPV over simple NCPAP for success in extubation when used as a bridge [21].
Finally, high-flow nasal cannula can deliver a small degree of CPAP and improvement in oxygenation, but the flow must always be humidified as this modality can quickly lead to significant mucosal drying and bleeding as well as a surprising degree of dehydration [22].
Invasive Modes
Once the decision has been made to provide invasive ventilation utilizing endotracheal intubation, there are a wide variety of ventilation options that can be utilized, with the ultimate goal of preventing damage to premature, underdeveloped lungs when exposed to prolonged positive pressure ventilation. Generally, the first choice ventilation strategy is the use of conventional ventilation, but often this proves difficult due to either the need for high inspiratory pressures, hypoxemia, or both. When this modality is not adequate, the two main alternatives are high-frequency oscillatory ventilation (HFOV) and high-frequency jet ventilation (HFJV).
A recent meta-analysis in Neonatology investigated the use of conventional ventilation (CV) versus HFOV, specifically looking at short-term mortality, chronic lung disease, and other forms of morbidity. They found no change in short-term (~30-day) mortality. They found more pulmonary air leaks with HFOV as well as increased rates of significant IVH with certain modes of HFOV, although this particular finding was not consistent. There was, however, a decrease in the risk of ROP in the HFOV group [23].
Conventional Ventilation
Conventional ventilation in neonates is not terribly different than CV in adults. Many of the same principles are utilized, such as lung-protective strategies and basic initial settings. One point that should be remembered is that lung-protective measures, such as low tidal volumes and peak inspiratory pressures, should be utilized on all premature neonates.
A recent meta-analysis suggests that using volume-targeted ventilation (as opposed to pressure-targeted ventilation) leads to decreased days on the ventilator, BPD, and significant IVH (grade 3 or 4) [24].
Time-cycled, pressure-limited ventilation (TCPL) is often utilized in neonates and is also known as intermittent positive pressure ventilation (IPPV). In this mode, the inspiratory and expiratory times are set, allowing many variables to affect the tidal volume with each breath, while providing a continuous background flow and limited pressure [20].
High-Frequency Oscillatory Ventilation
Instead of utilizing conventional ventilation to provide close to physiologic tidal volumes using large pressure differences, HFOV relies on a completely different strategy. HFOV starts by providing a constant distending pressure (mean airway pressure, or MAP) about which a rapidly oscillating pressure difference, or amplitude, occurs. In fact, this occurs so rapidly that the oscillating pressure wave is attenuated and the alveoli ultimately are exposed only to the MAP, or a very small pressure perturbation. This is thought to decrease the sheer stress, volutrauma, and barotrauma caused by large changes in pressure and distention experienced by alveoli during CV. Also, given the higher MAPs tolerated during HFOV, oxygenation is often vastly improved. Because the pressure difference is created by a piston driving an oscillating membrane back and forth to create the pressure change around the MAP, this is the only ventilation mode that utilizes an active expiratory phase.
With minimal changes in pressure seen by alveoli during HFOV, it is often not intuitive how gas exchange is accomplished. Gas exchange during HFOV seems to be accomplished via a variety of mechanisms, instead of the fairly simple bulk gas dispersion utilized by CV. A paper in Critical Care Medicine in 2005 sought to clarify these mechanisms, which include but are not limited to: molecular diffusion, turbulence, pendelluft, and laminar flow with Taylor dispersion [25].
The basic initial settings for HFOV involve setting a MAP, an amplitude (change in pressure around the MAP), a frequency (usually 8–15 Hz), and an FiO2. Unlike in conventional ventilation, where oxygenation and ventilation are somewhat coupled, when utilizing HFOV, oxygenation and ventilation are mostly determined by different variables. The MAP and FiO2 are the major determinants of oxygenation and the frequency and amplitude will largely determine the ventilation (with lower frequencies leading to increased ventilation and CO2 elimination). It is important to appreciate a “wiggle,” which is the subjective vibratory movement of the chest wall that occurs when there is an adequate pressure difference about the MAP. This is in fact how the amplitude is titrated to effect in neonatal HFOV. High-frequency oscillatory ventilation utilizes active exhalation, which means a negative pressure is applied to force volume out of the lungs.
High-Frequency Jet Ventilation
High-frequency jet ventilation is another form of high-frequency ventilation, which uses a combination of conventional ventilation and high-frequency pulses. The general setup is to have a conventional ventilator deliver positive end-expiratory pressure (PEEP) as well as a low respiratory rate of physiologic tidal volumes referred to as “sigh breaths.” Coupled to this is a jet injector that pulses short and rapid jets of gas into the trachea at a rate of 240–660 breaths per minute, although the usual operating range is more in the range of 320–450 breaths per minute. These jets entrain additional gas by creating an area of negative pressure, but do not employ active exhalation as seen in HFOV.
A Cochrane Database review looked at the elective use of HFJV versus CV in the population of preterm infants with RDS and found that those babies treated with HFJV had a lower incidence of chronic lung disease, but there was an unclear impact on the incidence of IVH [26].
Gastrointestinal
The gastrointestinal system undergoes a complex developmental process to ready the fetus for enteral nutrition at birth. Despite this, a disproportionate amount of the oxygen delivery in a fetus is directed toward the head, leaving the gut vulnerable to ischemic insults in general. In addition to this, development does not always proceed perfectly and can lead to congenital defects noted at birth. For more in-depth and comprehensive information, please read the chapter on gastrointestinal disorders.
Necrotizing Enterocolitis
Necrotizing enterocolitis (NEC) is bowel necrosis that occurs postnatally and is a significant source of morbidity for small and preterm infants, often carrying high mortality rates. NEC will classically present in a neonate with a variety of often subtle signs and symptoms, including feeding intolerance, apnea/bradycardia/desaturation events, abdominal distention, discoloration of the abdomen, peritonitis, and bloody stools. Abdominal radiographs may reveal pneumatosis intestinalis, free air, and portal venous gas in cases of severe or advanced NEC. The pathophysiology is not fully understood, but likely involves a combination of an immature gut with a delicate vascular supply, abnormal microbial colonization, immunoreactive mucosa, and a genetic predisposition. Treatment depends on the severity of illness and whether or not an intestinal perforation has occurred. The mainstay of treatment regardless of severity is a combination of bowel rest and intravenous antibiotics. For more severe cases a peritoneal drain may be placed at the bedside or an exploratory laparotomy with bowel resection may be performed, either at the bedside or in the operating room [27].
Omphalocele and Gastroschisis
Omphalocele (Figure 3.2) and gastroschisis (Figure 3.3) are both congenital abdominal wall defects that must be addressed early in the neonatal course and are often diagnosed prenatally. Beyond the fact that significant evaporative losses can occur with both, the details most important to the anesthesiologist regarding these conditions are the associated comorbidities. Associated congenital abnormalities are far more common in patients with omphaloceles than those with gastroschisis. In fact, cardiac anomalies occur in 18–24 percent of infants with an omphalocele, making a preoperative (and often fetal) echocardiogram an essential part of the evaluation of an infant with an omphalocele. The bowel in gastroschisis, however, does not have the benefit of a protective sac, as in omphalocele, so patients with gastroschisis often have significant degrees of bowel inflammation and subsequent strictures. Depending on the size of the defects, they may require staged closures, allowing the abdominal cavity time to grow and accept the gut without significant diaphragmatic competition and respiratory compromise [28].
Figure 3.2 Gastroschisis.
Figure 3.3 Omphalocele.
Jaundice
Given the typical hematologic and hepatic state of the normal newborn, it is not surprising that neonatal jaundice occurs, to some degree, in every infant. Neonatal jaundice refers to an elevated level of indirect (or unconjugated) bilirubin that is manifest by the yellow discoloration of the skin in a level-dependent progression from the tip of the nose, down the body (the further caudad the spread, the higher the level). Left untreated, severe hyperbilirubinemia can lead to an acute bilirubin encephalopathy, also known as kernicterus. The factors leading to significant jaundice include the large erythrocyte volume, the faster turnover of erythrocytes, and the decreased activity of uridine diphosphate glucuronosyl transferase, which is responsible for the conjugation of bilirubin into a water-soluble form that can be excreted by the kidneys.
Often, phototherapy is utilized to provide conjugation via ultraviolet energy so that excess bilirubin can be conjugated and excreted (Figure 3.4). This condition is self-resolving once liver enzymes become upregulated and red cell turnover diminishes. ABO incompatibility (where maternal antibodies attack neonatal erythrocytes) is one of the more common situations leading to severe hemolysis and jaundice in the newborn. Routine screening and surveillance has significantly decreased the morbidity of this “setup” for jaundice [29].
Fluid Management in the NICU
Perhaps the first thing a newcomer to the NICU will notice regarding fluid management is that it is reported as ml kg–1 day–1 and referred to as “total fluids” (TF). To the inexperienced, the selection of TF can seem random or arbitrary, but is based on a set of guiding principles.
Extremely premature infants are composed of almost entirely water, with a composition of water nearing 90 percent. These premature infants actually lose much more water transepidermally than their more mature counterparts, and this loss can quickly lead to dehydration. In fact, they can lose more water this way than through urine output. Many of the newer incubators incorporate variable humidification to counteract these losses in the first few days of life. Luckily, after birth, infants rapidly attain mature degrees of transepidermal losses and this is less of a concern with time.
The choices of TF as well as glucose and electrolyte additives must be managed carefully with frequent adjustments, at least on a daily basis. Smaller and earlier gestational age infants will require more fluids on a ml kg–1 day–1 basis, and this will often increase over the first few days of life. Modi suggests that a good starting point for an infant born at less than 1000 g is 100 ml kg–1 day–1, making adjustments based on strict inputs and outputs as well as weight and electrolyte measurements [30].
As infants progress through their first few days of life, they have a natural diuresis as well as tremendous metabolic requirement needed for growth and their high basal metabolic rate. Because of this, infants will need more and more fluid as the days progress. O’Brien and Walker outlined general guidelines for low birth weight (LBW) (<2.5 kg), very low birth weight (VLBW) (<1.5 kg), and extremely low birth weight infants (ELBW) (<1 kg). They suggest starting with 50–60 ml kg–1 day–1 and 80–90 ml kg–1 day–1 for LBW and VLBW/ELBW infants, respectively, on day of life 0–1, and increasing progressively to 150–180 ml kg–1 day–1 by age five days, although many units stop at the lower end of this range [31].
Many NICUs will use mild fluid restriction as a strategy to decrease the incidence of PDA, NEC, BPD, and IVH. A Cochrane meta-analysis published in 2008 found a significant decrease in incidence of PDAs and NEC in premature infants and a trend toward a decreased incidence of BPD formation and IVH. There was, however, a greater weight loss in premature infants who were fluid restricted and many of these infants required higher-density caloric feeds in order to achieve adequate growth. In addition, many NICUs will also treat patients with BPD with diuretic therapy, which has been shown to be helpful in BPD exacerbations. Many of these infants will require electrolyte replacement, especially if they are treated with loop diuretics. Anesthetizing these infants is a challenge because of their relatively dehydrated state. Carefully crafting an anesthetic to minimize intraoperative hypotension in order to decrease the need for fluid boluses is helpful. Educating NICU providers about the cardiac depressant and hypotensive effects of most general anesthetics and the resultant need for fluid resuscitation is essential to avoid conflicts about the fluid management of these vulnerable patients in the operating room.
Temperature Regulation
For the lay public, there is probably nothing more synonymous with premature infants than the incubator. As mentioned in the introduction, the invention of the neonatal incubator was the first step in the modern care of the neonate and in reality had a tremendous impact on neonatal mortality, especially in infants born at less than 1500 g. The neonate is accustomed to being in a warm intrauterine environment that is generally just a little warmer than the mother (a point often noted if a neonatal temperature is taken immediately after birth). Just like their adult counterparts, neonates attempt to maintain their core temperature within a narrow range, but they have far less ability to do so and have a massively increased potential for heat loss in comparison. However, unlike their older counterparts, neonates do not have the capability to shiver in their attempt to increase their temperature – they instead rely solely on the metabolism of brown fat, which is present in the neonate as early as 25 weeks GA. This vulnerability to temperature applies also to hyperthermia. In short, neonates depend on a neutral thermal environment, which is the range of temperatures in which a neonate must expend minimal energy in order to maintain an acceptable core temperature.
Infants, and especially LBW infants, have a much higher surface area to volume ratio, and therefore can quickly lose heat to the environment via radiation, convection, and conduction. Immediately following birth, the most important mechanism of heat loss is via evaporation – the newborn emerges wet and must be quickly dried to prevent these losses. In addition to this, the skin of a premature neonate is thin, with very little subcutaneous fat, both of which decrease the neonate’s insulating capacity. Small preterm infants are such poor insulators that simple measures such as placing the infant in a plastic bag or wrapping it in plastic wrap can greatly decrease heat loss.
As the infant’s temperature falls below 36°C, active rewarming should be initiated to prevent further drops in temperature. However, when the temperature falls below 32°C, one may anticipate severe metabolic, cardiac, and neurologic derangements and corrective actions should be taken immediately [32].
Neonatal Sepsis
Sepsis has been and continues to be a major source of morbidity and mortality for neonates, especially preterm neonates. Its presentation can be as overt or subtle as the presentation for NEC, and treatment must proceed immediately to prevent further insult or death. In fact, newborns will often react to bacteremia and sepsis with hypothermia, instead of pyrexia. Because neonatal sepsis can present in subtle ways and have devastating consequences, infants will often complete “rule-out sepsis” evaluations in which antibiotics are administered until surveillance body fluid cultures return negative at 48–72 hours. In addition, several biomarkers are gaining popularity to guide antibiotic treatment, including C-reactive protein and procalcitonin.
The organisms most commonly responsible for early-onset neonatal sepsis include, but are not limited to, group-B Streptococcus (GBS), Escherichia coli, Listeria, and Enterococcus. GBS infection was a significant pathogen in early neonatal sepsis that has been dramatically reduced with routine maternal GBS screening and treatment. GBS remains a concern in infants of inadequately treated mothers and later in infancy. Several neonatal sepsis evaluation algorithms exist, but most institutions in the United States utilize the guidelines distributed by the American Academy of Pediatrics in the Red Book [33].
Common Surgical Procedures in the NICU
The major surgical interventions for neonates are covered in great detail throughout this book. In this section, however, we will focus only on those procedures commonly performed in the NICU at the bedside. The procedure location is highly hospital-dependent, with some institutions opting to perform the majority of procedures within the NICU and others opting to transport patients to the operating room for the majority of procedures.
NEC Resection, Peritoneal Drain Placement
Exploratory laparotomies, intestinal resections, anastomosis, ostomy creations, and peritoneal drain placements are common in the NICU and, in fact, are the most common emergency surgical procedures performed on the neonatal population. It is more common among LBW and premature infants and presents in a myriad of subtle and overt ways. The choice of surgical procedure is highly dependent on the acuity of the infant, but it is well-recognized that infants requiring surgery of any kind have much higher morbidity and mortality [34].
PDA Ligation
When medical closure of a PDA is unsuccessful or inappropriate, surgical closure is often indicated. In fact, in cases of hemodynamically significant PDAs, surgical ligation is often the initial approach since it has a much higher success rate than indomethacin (or other cyclooxygenase inhibitors). The approach is through a left thoracotomy or very rarely thoracoscopically in larger infants.
The only other option is an endovascular closure in the cardiac catheterization lab, but this is generally only feasible for larger patients and not the neonatal population. Smaller devices are being investigated in animal models, with the hope of providing a safer and less expensive method of PDA closure than an operation [35].
A recent Cochrane review looked at the use of indomethacin for closure of a PDA versus a surgical closure and found no difference in any of the major morbidities queried, including mortality, NEC, sepsis, IVH, or CLD [36].
Operating in the OR vs. the NICU
Certain procedures will almost always require a trip to the operating room due to the need for specialized equipment, personnel, or lighting, or the increased degree of sterility, but many procedures can be safely performed within the NICU. Any trip to and from the operating room carries with it inherent risks of airway dislodgment, invasive line dislodgment, thermal disruption, hemodynamic instability outside of a direct clinical area, and interruption in current ventilatory strategies. There are well-founded arguments for and against each option.
This is not a new controversy; in fact, a paper in 1993 lays out nicely the arguments on each side. They compared the procedures performed in the NICU to those performed in the operating room over a four-year period and found that overall the patients managed in the NICU were of lower GA, lower weight, increased severity of illness, and far more likely to be mechanically ventilated preoperatively. There was a higher mortality among the patients treated in the NICU compared to the group brought to the operating room – this was attributed to the severity of illness and not surgical complications. Of note, they found no difference in sepsis (culture-proven), length of surgery, or vital sign stability, except for hyperthermia, which was higher in the operating room group [37].
In 2012, Hall et al. published their ten-year experience with performing operations in the NICU over a wide range of procedures, including many laparotomies, repairs of congenital diaphragmatic hernias, tracheostomies, and the insertion of central venous lines. They argue that such operations are safe and especially suitable for unstable and ventilated infants in order to avoid the risks associated with transport [38].
More recently, a point–counterpoint was outlined in the journal Pediatric Anesthesia. To summarize, the group feeling that it is best to travel to the operating room seems to be working from the construct that whatever makes the surgical team more comfortable, safe, and efficient must be what is best for the infant. They focus on issues such as resource familiarity and an aseptic environment. On the other hand, the group arguing for more operations occurring in the NICU seems to work from a construct of focusing on the neonate and minimizing transfers, interruptions in therapies, and physiologic perturbations. They feel that sick neonates should remain in place, and we, as providers, should be more flexible and accommodating in order to achieve this [39].
This controversy continues and does not appear to have an easy answer. Individual institutions will continue to have their preferences, but what is most important is to recognize when these preferences should be altered. For instance, in an institution where all neonatal surgery is performed in the operating room, there may be instances in which the transport is simply too precarious and an exception must be made. Conversely, in institutions frequently performing operations in the NICU, there may be circumstances in which it is more prudent to transport to the operating room. As in most areas of medicine, flexibility is vital in the care of our sickest, most vulnerable patients.
Postoperative Care in the NICU
Prevailing thought regarding pain in the neonate has shifted drastically over the past century. Initially, many felt that the neonatal nervous system was not adequately mature to experience pain; while this may seem barbaric by today’s standards, it certainly guided practice in the not too distant past. There is overwhelming evidence that even the smallest of neonates are capable of experiencing pain and discomfort, but what may be more important is newly emerging evidence that painful experiences may have consequences for years to come.
In 1987, Anand et al. published a study investigating the adjunctive use of fentanyl in neonates undergoing PDA ligation, in addition to the standard of care at the time, which was a mixture of oxygen and nitrous oxide. They observed significant physiologic changes in the non-fentanyl group in response to pain, including changes in cardiorespiratory, metabolic, and hormonal systems [40].
Later work has further clarified these acute physiologic alterations, which can in themselves have a significant impact on the neonate. In addition to these physiologic changes, infants will often display behavioral alterations in response to pain. Collectively, the behavioral and physiologic changes form the basis for neonatal pain scales.
Neonatal pain pathways are still in a state of development, so there is growing concern that painful stimuli, especially if repeated, could lead to desensitization and hyperalgesia. One of the more famous investigations centered on neonates who had received circumcisions without analgesia, and showed significantly altered behavior with subsequent vaccinations months later [41]. There is also evidence that surgery increases the degree of sensory processing dysfunction that occurs in children who have spent time in the NICU as premature neonates [42–44].
Pain control in the immediate postoperative period is of paramount importance and intraoperative strategies and observations should be communicated clearly with the NICU team in order to develop an appropriate postoperative analgesic regimen. Many units have created analgesic guidelines for common invasive procedures in the NICU, including the perioperative period.
Ethics in the NICU
As in any critical care setting, ethical dilemmas are not infrequent, and the NICU is no exception. While it is clear that neonates lack the capacity to make decisions for themselves, there is no ethical basis for limiting their right to dignity, especially at the end of life. The tremendous improvements in neonatal care have allowed a survival rate approaching 80 percent for extremely premature infants. What this brings with it, though, are more complex ethical scenarios centering on quality of life and the rights of the newborn [45].
Threshold of Viability
Within the past few decades, the mortality for extremely premature infants has fallen from ~80 percent to ~20 percent, but significant morbidities persist. We also lack consensus as to the definition of the “threshold of viability.”
Certain seminal cases highlight the ethical dilemmas present when the subject is a patient who may or may not be a viable human being, depending on who one asks. In Miller v. Hospital Corporation of America, the Miller family sued the hospital organization for resuscitating their baby at less than 24 weeks GA despite their wishes to withhold resuscitation. This infant survived, but with profound neurologic deficits. The courts initially found in the family’s favor, but they lost in appeals at the level of the Supreme Court of Texas on the grounds that parents cannot be fully informed as to the infant’s prognosis until after birth, and could therefore not prenatally refuse resuscitation. This was further evaluated by Robertson in the 2004 Hastings Center Report, where the argument was made that parents could refuse life-saving treatment, but only if it was unlikely that the child could later interact symbolically [46,47].
A recent study looked at the guidelines for resuscitation at the threshold of viability in many countries. There was strong consensus that there is no chance for survival at less than 22 weeks GA, but at 25 weeks GA and above, resuscitation is generally offered except in cases of severe congenital abnormalities. Most countries consider the time between 23 and 24 weeks GA to be murkier, and these infants are approached on a more individual basis [48].
In the United States, the American Academy of Pediatrics published a clinical report addressing infants born between 22 and 25 weeks GA. They argue for full disclosure to the parents and a team approach to decision-making, including the parents. Furthermore, they recommend respecting the parents’ wishes if found to be “within the limits of medical feasibility and appropriateness” [49].
Conclusions
The NICU is a dynamic environment characterized by unique patients, comorbidities, treatment modalities, and technology. It is staffed by a highly trained team with exquisite attention to detail. The pediatric anesthesiologist and neonatologist arrive at their professions via somewhat different paths, but their understanding of one another’s role and environment is critical to the optimal care of the neonate.
References
The physiologic changes that occur at birth and continue over the first few days and the first months of life are considerable and need to be appreciated in order to understand the hemodynamics of newborns.
Fetal Circulation
Fetal circulatory channels shunt blood away from the lung, such that both ventricles, in parallel, contribute to systemic oxygen delivery by pumping blood to the systemic arterial system. This parallel circulation permits normal fetal growth and development even in fetuses with cardiac malformations.
Oxygenated blood from the placenta returns to the fetus via the umbilical vein, which enters the portal venous system. The ductus venosus connects the left portal vein to the left hepatic vein at its junction with the inferior vena cava (IVC). This allows approximately 50 percent of umbilical venous blood to bypass the hepatic sinuses. The remainder of the umbilical venous flow passes through the liver and enters the IVC via the hepatic veins. Fetal IVC blood is a combination of blood from the lower fetal body, umbilical vein, and hepatic veins. The stream of blood from the ductus venosus has a higher velocity in the IVC than the stream from the lower body and hepatic veins. This higher velocity facilitates delivery of this higher oxygen content blood across the foramen ovale (FO) into the left atrium (LA) [1] (Figure 4.1).
Inferior vena cava blood enters the right atrium (RA) and due to the position of the Eustachian valve, Chiari network, and FO enters the LA during 80 percent of the cardiac cycle. During the other 20 percent (atrial systole), IVC blood crosses the tricuspid valve and enters the right ventricle (RV). The overwhelming majority of superior vena cava (SVC) crosses the tricuspid valve and enters the RV as well. Blood from the RV is ejected into the pulmonary artery (PA). Approximately 10–15 percent of blood from the PA passes through the lungs to reach the LA and the rest is shunted to the distal aorta via the ductus arteriosus (DA). As a result, two-thirds of total fetal cardiac output is provided by the RV with the remaining one-third provided via the LV.
The dynamics of shunting at the level of the ductus venosus, FO, and DA result in preferential delivery of the most highly oxygenated blood to the coronary and cerebral circulations. Obviously, this preferential delivery of oxygenated blood may be compromised in utero by cardiac lesions that prevent or reduce LV output. At birth, a series circulation is established in which each ventricle pumps into a specific vascular bed (RV to pulmonary artery; LV to aorta). The removal of the placenta and the initiation of alveolar ventilation at birth have the immediate effect of establishing this series circulation. To maintain the adult series circulation, the fetal channels must be closed (Table 4.1). Complex neurochemical and hormonal influences affect the closing of these fetal shunts. Acidosis, sepsis, hypothermia, hypoxia, and hypercarbia may cause reopening of the shunts and persistence of the fetal circulation (PFC).
Table 4.1 Fetal structures and their corresponding structure in adults
Fetal structure | Adult structure |
---|---|
Foramen ovale | Fossa ovalis |
Umbilical vein | Ligamentum teres |
Ductus venosus | Ligamentum venosum |
Umbilical arteries | Medial umbilical ligaments, superior vesicular artery |
Ductus arteriosus | Ligamentum arteriosum |
Closure of the Ductus Arteriosus
In the fetus, patency of the ductus arteriosus is maintained by high levels of prostaglandin (PGI2 and PGE1). There are two stages of ductal closure in the newborn: functional closure and permanent anatomic closure. Functional closure occurs by contraction of the smooth muscle of the ductal wall, usually within the first day of life. An increase in PO2 and a decrease in prostaglandins contribute to functional closure. Oxygen is a dose-dependent ductal constrictor that acts by increasing the rate of oxidative phosphorylation within smooth muscle cells. In addition, the response to oxygen may be age-related; full-term neonates have a more dramatic response to oxygen than do immature newborns. Norepinephrine and epinephrine, by changing pulmonary and systemic vascular resistances, may secondarily contribute to ductal closure. Acetylcholine has a direct constrictor effect on ductal tissue. Permanent anatomic closure of the duct is usually accomplished by 2–3 weeks of life in the normal full-term neonate. The lumen is sealed by fibrous connective tissue, leaving the vestigial structure, known as the ligamentum arteriosum.
Closure of the Foramen Ovale
In utero, the RA pressure is higher than the LA pressure. Inferior vena cava blood flows in such a manner as to keep the FO open. Cessation of umbilical vein flow causes a significant decrease in venous return to the right heart, causing a decrease in RA pressure. In addition, ventilation causes a marked increase in pulmonary arterial and venous blood flow, resulting in an increase in LA pressure. This elevation of LA relative to RA pressure causes the flap-like valve of the FO to functionally close. In instances in which RA pressure remains elevated, right-to-left shunting may persist. Functional closure usually progresses to anatomic closure. However, probe patency of the FO may persist in 30 percent of normal adults and 50 percent of children younger than five years of age.
Closure of the Ductus Venosus
The umbilical vessels constrict strongly after mechanical stimulation and high oxygen tension facilitate this process. The resultant decrease in umbilical venous blood flow causes passive closure of the ductus venosus. The ductus venosus does not appear to be as sensitive as the ductus arteriosus to PaO2, PaCO2, or pH. The ductus venosus is functionally closed by one week of life and anatomically closed by three months. The remaining structure is the ligamentum venosum.
In addition to the establishment of the adult series circulation, dramatic alterations in pulmonary circulation, cardiac output and distribution, myocardial performance, and myocardial cell growth and hypertrophy continue to occur during the first weeks, months, and even years of life.
Pulmonary Vascular Changes
The fetus has low pulmonary blood flow secondary to a high pulmonary vascular resistance. The minimal blood flow that reaches the pulmonary bed has a very low PaO2, which may cause hypoxic pulmonary vasoconstriction and contributes to the elevated pulmonary resistance seen in the fetus. Morphologic examinations of the small arteries of the fetal and newborn lung show a thick medial smooth muscle layer. The fetal pulmonary vasculature is reactive to a number of stimulants. Vasoconstriction is induced by decreases in PaO2, pH, and leukotrienes. Acetylcholine, histamine, bradykinin, PGE1, PGE2, PGI2 (prostacyclin), and prostaglandin D2 and beta-adrenergic catecholamines are patent vasodilators of fetal pulmonary vessels.
At birth, alveolar ventilation commences. This reduces the mechanical compression of small pulmonary vessels and increases PaO2. The result is a dramatic reduction in pulmonary vascular resistance (PVR). During the following weeks and months, remodeling of the pulmonary vessels occurs; the most notable change is a thinning of the medial smooth muscle layer. By six months of life, this process results in reduction of PVR to near normal adult levels. The normal process of postnatal pulmonary maturation may be altered significantly by pathologic conditions, such as those associated with congenital heart disease.
Myocardial Performance in the Neonate
In utero, the right ventricle (RV) has a cardiac output of approximately 330 ml kg–1 min–1 compared with the left ventricular (LV) output of 170 ml kg–1 min–1. Table 4.2 summarizes the percentage of combined CO at different gestational ages [2]. At birth, both RV and LV eject an output of approximately 350 ml kg–1 min–1. This requires minimal stroke volume increase for the RV but a considerable increase in stroke volume for the LV. The high-output state of the newborn effectively limits further increases in cardiac output. This high-output state decreases to about 150 ml kg–1 min–1 by 8–10 weeks of life.
Table 4.2 Percentage of combined cardiac output at different gestational age
20 weeks | 30 weeks | 38 weeks | |
---|---|---|---|
Combined CO | 210 ml min–1 | 960 ml min–1 | 1900 ml min–1 |
Left ventricle | 47 percent | 43 percent | 40 percent |
Right ventricle | 53 percent | 57 percent | 60 percent |
Data extrapolated from [2].
Hemodynamic Changes at Birth
Myocardial morphology and performance is notably different in the neonate. These differences are listed in Table 4.3 and summarized as follows.
Table 4.3 Neonatal myocardial performance compared to the adult
Afterload mismatch |
Limited preload reserve |
Reduced contractile capacity |
Reduced ventricular compliance |
Increased intraventricular dependence |
Incomplete autonomic sympathetic innervation and dominance of parasympathetic |
Immature myocardial metabolism |
Afterload Mismatch. The neonatal heart is more susceptible to afterload mismatch, and therefore stroke volume is poorly maintained in the face of increasing outflow resistance.
Limited Preload Reserve. The neonatal heart has limited preload reserve. Augmentation of stroke volume via the Frank–Starling mechanism is limited as compared with an adult.
Reduced Contractile Capacity. Neonatal cardiac cells contain more water and fewer contractile elements than mature myocardium. In addition, there are fewer mitochondria and sarcoplasmic reticulum (SR) and poorly formed T-tubule that make the myocardium more dependent on extracellular calcium. Development of the SR, T-tubular system, and calcium-handling proteins appear to be rapid and it has been suggested that they are relatively mature by three weeks in the neonatal heart [3].
Reduced Ventricular Compliance. The compliance of the neonatal myocardium is reduced because a deficiency of elastic elements parallels the deficiency of contractile elements.
Increased Intraventricular Dependence. Changes in ventricular pressure are transmitted to the opposite ventricle via the ventricular septum more readily in the immature myocardium. Left ventricular diastolic filling is disproportionately impaired in the neonate by high RV end-diastolic pressure. This is due to a leftward shift of the intraventricular septum and a reduction in LV distensibility. Right ventricular diastolic filling is impaired to an equal extent by high LV end-diastolic pressure in neonates. This enhanced ventricular interaction is caused by reduced ventricular compliance and because, at birth, the LV and RV are of equal mass. The increased volume and pressure load experienced by the LV after birth produces relative LV hypertrophy. The normal adult LV to RV mass ratio of 2:1 is not seen until several months after birth.
Incomplete Autonomic Innervation. Sympathetic innervation, which is responsible for increasing heart rate and contractility, is incompletely developed at birth. As a result, local myocardial release of norepinephrine contributes less to increases in contractility than do increases in circulating catecholamine levels. For this reason, inotropic agents such as dopamine, the effects of which are partially mediated through release of norepinephrine from myocardial nerve endings, may have to be used in higher doses to be effective in younger patients. On the other hand, the parasympathetic system, which reflexly slows the heart, is fully functional at birth.
Immature Myocardial Metabolism. The neonatal myocardium is more dependent on anerobic metabolism than the adult heart using carbohydrates and lactate as primary energy sources. This may have a somewhat protective effect, making the neonatal myocardium more tolerant to the effects of hypoxia.
Defining Hypotension
Defining hypotension in preterm and term neonates is problematic. As a consequence, there are multiple definitions of hypotension, incorporating both mean and systolic blood pressure, defined in the pediatric anesthesia and neonatology literature. The Pediatric Advanced Life Support definition of neonatal hypotension is a systolic blood pressure (SBP) less than 60 mmHg. A survey of members of the Society of Pediatric Anesthesia and the Association of Paediatric Anaesthetists identified an SBP of 45–50 mmHg as the threshold value for hypotension in neonates [4]. A mean arterial blood pressure (MAP) less than 20–30 percent of baseline is considered hypotension by many providers. In general, neonatologists define hypotension as blood pressure below the fifth or tenth percentile for gestational and postnatal age. A consensus statement addressing the lower limit of MAP by the Joint Working Group of the British Association of Perinatal Medicine and the Research Unit of the Royal College of Physicians recommended that MAP should not drop below the infant’s gestational age in weeks, which also happens to be very close to the tenth percentile for age [5]. An absolute lower limit for mean blood pressure of 30 mmHg in infants <30 weeks’ postmenstrual age has also been suggested because a MAP <30 mmHg is associated with lower cerebral blood flow and cerebral injury [6]. Defining normal BP ranges in very low birth weight (VLBW, <1500 g) and extremely low birth weight (ELBW, <1000 g) preemies is even more complicated by the fact that ductual closure and transition from fetal circulation may be delayed. Nonetheless, BP ranges based on weight and gestational age have been defined [7–9] (Table 4.4; Figure 4.2)
Table 4.4 Blood pressure in premature infants: birth weight 600–1750 g with (2 × SD)
Age(days) | Wt (g) | |||||||
---|---|---|---|---|---|---|---|---|
600–999 | 1000–1249 | 1250–1499 | 1500–1750 | |||||
sys | dia | sys | dia | sys | dia | sys | dia | |
1 | 39(17) | 23(10) | 44(23) | 23(14) | 48(18) | 27(12) | 47(16) | 26(16) |
3 | 45(16) | 31(12) | 48(15) | 37(10) | 59(21) | 40(14) | 51(18) | 35(10) |
7 | 50(15) | 30(12) | 57(14) | 43(17) | 68(15) | 40(11) | 66(23) | 41(24) |
14 | 50(15) | 37(12) | 53(30) | 64(21) | 36(24) | 76(35) | 42(20) | |
28 | 61(24) | 46(27) | 57(30) | 69(31) | 44(26) | 73(6) | 50(10) |
Data from Handbook of Anesthesiology, Department of Anesthesiology, Perioperative and Pain Medicine, Boston Children’s Hospital.
Figure 4.2 The blood pressure increases over the first week of life and the slope of increase is similar irrespective of gestational age.
In addition to the difficulty of defining normal blood pressure, anesthesiologists must consider the limitations of obtaining an accurate blood pressure in neonates (Chapter 20). In normal, healthy, awake neonates, there is a wide variation in blood pressures between limbs (>20 mmHg) as shown by Crossland et al. [10]. It is also important to note that treating hypotension with inotropic support is a challenge in neonates. The neonatal myocardium is vulnerable to the effects of catecholamines in terms of myocardial damage and depletion of energy substrates [11].
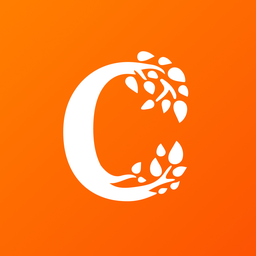
Full access? Get Clinical Tree
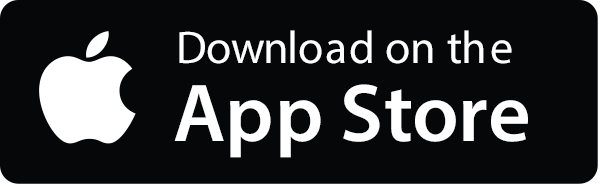
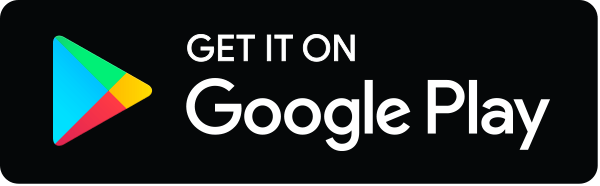
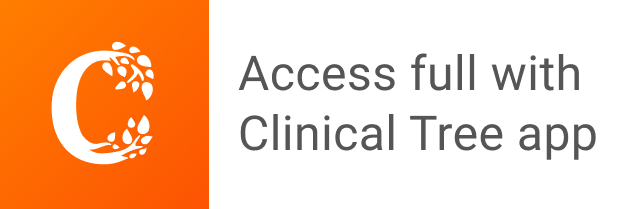