Routine Monitoring of Critically Ill Patients
Alan Lisbon
Michael D. Howell
Frederick J. Curley
Nicholas A. Smyrnios
The ability to intensively, continuously, and routinely monitor both usual vital signs and more specialized metrics forms one of the cornerstones of modern critical care. One of the key differences between intensive care units (ICUs) and other hospital units is the level of detail with which patients are routinely monitored. This careful monitoring alerts the health care team to changes in the patient’s severity of illness—helping both to diagnose disease and assess prognosis. Careful monitoring also helps the health care team safely apply therapies such as volume resuscitation, vasoactive infusions, and mechanical ventilation.
This chapter deals with the routine and predominantly noninvasive monitoring that should be done for most patients in critical care units. It examines the indications for, the fundamental technology of, and the problems encountered in the routine monitoring of temperature, blood pressure, electrocardiographic (ECG) rhythm, ST segments, respiratory rate/pattern, and oxygen and carbon dioxide levels. In addition, it reviews noninvasive monitoring of tissue perfusion, with particular attention to gastric tonometry, sublingual capnometry, and trans-cutaneous oxygen and carbon dioxide monitoring.
Types of Monitoring Systems
When ICUs came into being in the late 1950s, nurses monitored patients’ vital signs intermittently. Continuous measurement was either unavailable or necessitated invasive procedures. The explosion in the use of technology during the past few decades has significantly changed critical care. All routine vital signs can now be monitored accurately, noninvasively, and continuously. As a result, today’s patients are monitored more intensively and continuously in the ICU than in any other part of the hospital, with the possible exception of the operating room.
During the past decades, the trend in monitoring systems has been toward multipurpose systems that monitor a variety of parameters. Commercial systems now monitor multiple invasive and noninvasive blood pressures, core temperature, respiration, oxygenation, end-tidal carbon dioxide, central and mixed venous oxygen saturations, ECG rhythm, and ST segments. Multipurpose systems eliminate the need for multiple, freestanding devices, which also reduces clutter and improves workflow ergonomics at the bedside. They can coordinate the monitoring of different parameters and manage data from other sources such as a ventilator or infusion pump. These multipurpose monitoring systems also work in conjunction with commercially available critical care information systems to provide more efficient data management, quality assurance reports, and, in some cases, prospective data-driven alerts.
Monitoring systems for ICUs may be either configured or modular. Configured systems have all of their functions hardwired directly into the system. Modular systems have removable modules that interface with the monitor and contain information for each parameter to be monitored. Modular systems have gained popularity as the physiologic parameters being monitored have changed and expanded. Modular systems can be upgraded more easily than hardwired systems.
Temperature Monitoring
Temperature changes in the critically ill patient are associated with significant morbidity and mortality [1, 2], making it clinically important to recognize an abnormal temperature. In one surgical ICU study, rectal temperatures on admission were normal in only 30% of patients, were above 37.6°C in 38%, and were below 36.8°C in 32% [3]. An abnormal temperature is frequently the earliest clinical sign of infection, inflammation, central nervous system dysfunction, or drug toxicity. Unfortunately, the type of thermometer and the site where the temperature is taken can affect the accuracy of this vital measurement. Clinicians should understand the impact of the thermometer type and the measurement site on obtaining an accurate and responsive estimation of the patient’s core temperature.
Types of Thermometers
Mercury-in-Glass Thermometers
Although mercury-in-glass thermometers have historically been the most common type in clinical use, environmental and health concerns related to mercury have resulted in several state and local legislative efforts to phase out this type of thermometer [4, 5]. Mercury and other liquid-expansion-based thermometers can give a falsely low measurement when the thermometer is left in place for less than 3 minutes; falsely high temperatures result from failure to shake the mercury down. Because of practical
limitations in length, different models of mercury thermometers are used for the hypothermic temperature range.
limitations in length, different models of mercury thermometers are used for the hypothermic temperature range.
Liquid Crystal Display Thermometers
Liquid crystal display (LCD) thermometers contain liquid crystals embedded in thin adhesive strips that are directly attached to the patient’s skin. LCD thermometers can be applied to any area of the skin but are most commonly applied to the forehead for ease of use and steady perfusion. Like all skin temperature measurements, they may poorly reflect core temperature when the skin is hypoperfused or patients have vasomotor instability. Forehead skin temperature is typically lower than core temperatures by 2.2°C [6], and changes in LCD forehead temperature lag behind changes in core temperature by more than 12 minutes [7]. LCD skin thermometry is probably best used in patients with stable, normal hemodynamics who are not expected to experience major temperature shifts and in whom the trend of temperature change is more important than the accuracy of the measurement.
Standard Digital Thermometers: Thermocouples and Thermistors
Electric thermometers that convert the electrical temperature signal into digital displays frequently use thermocouples and thermistors as probes. Thermocouples and thermistors can be fashioned into thin wires and embedded in flexible probes that are suitable for placing in body cavities to measure deep temperature.
Thermocouples consist of a tight junction of two dissimilar metals. The voltage change across the junction can be precisely related to temperature. The measuring thermocouple must be calibrated against a second constant-temperature junction for absolute temperature measurements. The measured voltage changes are on the order of 50 μV per degree Celsius and must be amplified to generate a usable temperature display. In the range of 20°C to 50°C, thermocouples may have a linearity error of less than 0.1 [8].
Thermistors consist of semiconductor metal oxides in which the electrical resistance changes inversely with temperature. A linearity error of up to 4°C may occur over the temperature range of 20°C to 50°C, but this can be substantially reduced by making mathematical adjustments or placing a fixed resistance in parallel with the thermistor, which decreases its sensitivity and usable temperature range [8]. Thermistors are more sensitive, faster responding, and less linear than thermocouples or semiconductors [8, 9]. Semiconductors measure temperature by taking advantage of the fact that the base-to-emitter voltage change is temperature-dependent, whereas the collector current of the silicon resistor is constant.
Tympanic Infrared Emission Detection Thermometers
Commonly used in a hospital setting, infrared emission-detection tympanic thermometers use a sensor that detects infrared energy emitted by the core temperature tissues behind the tympanic membrane [10]. The infrared emissions through the tympanic membrane vary linearly with temperature. The thermometer’s sensor sends a signal to a microprocessor, which converts the signal into a digitally displayed temperature. Measurements are most accurate when the measuring probe blocks the entrance of ambient air into the ear canal and when the mid-posterior external ear is tugged posterosuperiorly so as to direct the probe to the anterior, inferior third of the tympanic membrane. Operator error due to improper calibration, setup, or poor probe positioning can significantly alter temperatures [11]. However, a study controlling for these factors still found that tympanic temperatures frequently disagree with pulmonary artery temperatures in critically ill patients. In this study, more than 25% of tympanic temperature measurements differed by more than 0.6°C.
Temporal Artery Infrared Emission Detection Thermometers
More recently, infrared technology has also been used to noninvasively measure temperature over the temporal artery. Using these devices, a probe is passed over the forehead and searches for the highest temperature; some systems also scan the area behind the ear. An algorithm that estimates ambient heat loss and blood cooling then estimates the core temperature. The device is convenient, painless, and provides a rapid reading. One study of 57 critically ill adults found good correlation with pulmonary artery temperatures. However, patients in this study were normothermic and the authors concluded that these results should not be extrapolated to hypo- or hyperthermic patients [12]. In another study in patients with a broader temperature range, 89% of measurements differed from pulmonary artery temperatures by more than 0.5°C, the amount the authors had specified a priori as clinically significant [13]. Similar results have been found in febrile children [14]. Although comparatively few studies have assessed this method, it appears that temporal artery temperature measurements may differ significantly from measured core temperature in febrile or hypothermic critically ill patients.
Measurement Sites
The goal of temperature measurements is generally to estimate core temperature, the deep body temperature that is carefully regulated by the hypothalamus so as to be independent of transient small changes in ambient temperature. Core temperature exists more as a physiologic concept than as the temperature of an anatomic location. An ideal measurement site would be protected from heat loss, painless and convenient to use, and would not interfere with the patient’s ability to move and communicate. No one location provides an accurate measurement of core temperature in all clinical circumstances.
Sublingual Temperature Measurements
Sublingual temperature measurements are convenient, but suffer numerous limitations. Although open- versus closed-mouth breathing [15] and use of nasogastric tubes do not alter temperature measurement [16], oral temperature is obviously altered if measured immediately after the patient has consumed hot or cold drinks. Falsely low oral temperatures may occur because of cooling from tachypnea [17]. Sixty percent of sublingual temperatures are more than 1°F lower than simultaneously measured rectal temperatures; 53% differ by 1°F to 2°F, and 6% differ by more than 2°F. Continuous sublingual measurement interferes with the patient’s ability to eat and speak, and it is difficult to maintain a good probe position. Sublingual measurement is best suited for intermittent monitoring when some inaccuracy in measurement can be tolerated.
Axillary Temperature Measurements
Axillary temperatures have been used as an index of core temperature and can be taken with a mercury-in-glass thermometer, a flexible probe, or an LCD thermometer. Although some studies indicate close approximation of the axillary site with pulmonary artery temperatures [12], temperatures average 1.5°C to 1.9°C lower than tympanic temperatures [18]. Positioning the sensor over the axillary artery may improve accuracy. The accuracy and precision of axillary temperature measurements are less than at other sites [18], perhaps due in part to the difficulty of maintaining a good probe position.
Rectal Temperature Measurements
Rectal temperature is measured with a mercury-in-glass thermometer or a flexible temperature sensor. It is clearly the most widely accepted standard of measuring core temperature in clinical use. Before a rectal thermometer is inserted, a digital rectal examination should be performed because feces can blunt temperature measurement. Readings are more accurate when the sensor is passed more than 10 cm (4 inches) into the rectum [9]. Rectal temperature correlates well in most patients with distal esophageal, bladder, and tympanic temperatures [18, 19]. Rectal temperatures typically responds to induced changes in temperature more slowly than other central measurement sites [20, 21].
Esophageal Temperature Measurements
Esophageal temperature is usually measured with an electric, flexible temperature sensor. On average, esophageal temperatures are 0.6°C lower than rectal temperatures [22]. However, the measured temperature can vary greatly depending on the position of the sensor in the esophagus. In the proximal esophagus, temperature is influenced by ambient air [23]. During hypothermia, temperatures in different portions of the esophagus may differ by up to 6°C [23]. Stable, more accurate temperatures are reached when the sensor is 45 cm from the nose [23, 24]. Because of the proximity of the distal esophagus to the great vessels and heart, the distal esophageal temperature responds rapidly to changes in core temperature [9]. Changes in esophageal temperature may inaccurately reflect changes in core temperature when induced temperature change occurs because of the inspiration of heated air, gastric lavage, or cardiac bypass or assist [9].
Tympanic Temperature Measurements
Health care providers must measure tympanic temperature with specifically designed thermometers that are commonly used in the ICU. However, several studies have questioned their reliability in estimating ICU patients’ core temperatures [25, 26, 27, 28 and 29]. Accuracy depends in part on operator experience; however, one study showed that even when trained and experienced ICU nurses use tympanic thermometers, the variability in repeated measurements was more than 0.5°F in 20% of patients [30]. Unlike temporal artery measurements, which are not known to have complications, tympanic temperature measurements come with some risk. Perforation of the tympanic membrane [31] and bleeding from the external canal due to trauma from the probe have been reported.
Temporal Artery Measurements
Temporal artery measurements are not known to have complications. Their accuracy is reviewed in a previous section.
Urinary Bladder Temperature Measurements
Providers can easily measure the urinary bladder temperature with a specially designed temperature probe embedded in a Foley catheter [18, 19, 20 and 21]. In patients undergoing induced hypothermia and rewarming, bladder temperatures correlate well with great vessel and rectal temperatures and less well with esophageal temperatures [19, 20 and 21]. Bladder temperature under steady-state conditions is more reproducible than that taken at most other sites [19].
Central Circulation Temperature Measurements
ICU practitioners can measure the temperature of blood in the pulmonary artery using a thermistor-equipped pulmonary artery catheter. The temperature sensor is located at the distal tip and can record accurate great vessel temperatures once the catheter is in place in the pulmonary artery. Pulmonary artery temperatures are generally accepted as the gold standard for accurate measures of core temperature, although readings might be expected to differ from core temperature when heated air is breathed or warm or cold intravenous fluids are infused. Inserting a central venous thermistor specifically to monitor temperature is probably warranted only when other sites are believed to be unreliable, and when accurate, rapid, continuous temperature measurements are critical to the patient’s management.
Selecting the Measurement Site
The site used to monitor temperature must be an individualized choice, but certain generalizations can be made. Bladder [19, 32], esophageal, and rectal temperatures in general appear to be most accurate and reproducible [33], although rectal temperatures may lag behind other temperatures when the patient’s status is changing quickly. Because esophageal, rectal, and bladder sites appear similar in performance, convenience may help dictate appropriate site selection. Routine measurement of esophageal temperatures would necessitate inserting an esophageal probe in patients. In addition, because small changes in position can affect the accuracy of the measurement, routine esophageal temperature measurement is probably of benefit only in patients undergoing treatment for hyperthermia or hypothermia. Meanwhile, rectal probes may be extruded and may be refused by patients. Reusable, electronic, sheath-covered rectal thermometers have been associated with the transmission of Clostridium difficile and vancomycin-resistant Enterococcus [34, 35]. The third option, bladder temperature monitoring, is simplified by the fact that most critically ill patients have an indwelling Foley catheter. Monitoring the bladder temperature in these patients requires only a thermistor-equipped catheter. Patients with a thermistor-tipped pulmonary artery catheter already in place require no additional monitoring.
Indications for Temperature Monitoring
The Society of Critical Care Medicine’s Task Force on Guidelines’ recommendations for care in a critical care setting grades temperature monitoring as an essential service for all critical
care units [36]. Critically ill patients are at high risk for temperature disorders because of debility, impaired control of temperature, frequent use of sedative drugs, and a high predisposition to infection. All critically ill patients should have core temperature measured at least intermittently. Patients with marked temperature abnormalities should be considered for continuous monitoring; patients undergoing active interventions to alter temperature, such as breathing heated air or using a cooling-warming blanket, should have continuous monitoring to prevent overtreatment or undertreatment of temperature disorders.
care units [36]. Critically ill patients are at high risk for temperature disorders because of debility, impaired control of temperature, frequent use of sedative drugs, and a high predisposition to infection. All critically ill patients should have core temperature measured at least intermittently. Patients with marked temperature abnormalities should be considered for continuous monitoring; patients undergoing active interventions to alter temperature, such as breathing heated air or using a cooling-warming blanket, should have continuous monitoring to prevent overtreatment or undertreatment of temperature disorders.
Arterial Blood Pressure Monitoring
The first recorded blood pressure measurement occurred in 1733 and, somewhat surprisingly, was intraarterial pressure monitoring. The Reverend Stephen Hales placed a 9-foot brass tube in a horse’s crural artery and found a blood pressure of about 8 feet 3 inches. This was obviously not clinically applicable. In the mid-1800s, Carl Ludwig recorded the first arterial pressure waveforms, but it was not until 1881 that the first noninvasive blood pressure recordings were made. In 1896, Riva-Rocci developed and popularized the mercury sphygmomanometer, which was then adopted and disseminated at least in part by Harvey Cushing. In 1905, Korotkoff developed techniques for detecting diastolic pressure by listening for what are now called Korotkoff sounds [37]. More clinical techniques of direct blood pressure measurement by intraarterial cannula were initially developed in the 1930s and popularized in the 1950s [38, 39]. These measurements were soon accepted as representing true systolic and diastolic pressures.
Since that time, a variety of invasive and alternative indirect methods have been developed that equal and even surpass auscultation in reproducibility and ease of measurement. This section examines the advantages and disadvantages of various methods of arterial pressure monitoring and provides recommendations for their use in the ICU.
Noninvasive (Indirect) Blood Pressure Measurement
Providers can indirectly monitor blood pressure using a number of techniques, most of which describe the external pressure applied to block flow to an artery distal to the occlusion [40, 41, 42, 43 and 44]. These methods therefore actually detect blood flow, not intraarterial pressure [40], although one method describes the pressure required to maintain a distal artery with a transmural pressure gradient of zero. These differences in what is actually measured are the major points of discrepancy between direct and indirect measure-ments.
Indirectly measured pressures vary depending on the size of the cuff used. Cuffs of inadequate width and length can provide falsely elevated readings. Bladder width should equal 40% and bladder length at least 60% of the circumference of the extremity measured [45]. Anyone who makes indirect pressure measurements must be aware of these factors and carefully select the cuff to be used.
Manual Methods
Auscultatory (Riva-Rocci) Pressures
The traditional way to measure blood pressure involves inflating a sphygmomanometer cuff around an extremity and auscultating over an artery distal to the occlusion. Sounds from the vibrations of the artery under pressure (Korotkoff sounds) indicate systolic and diastolic pressures [43]. The level at which the sound first becomes audible is taken as the systolic pressure. The point at which there is an abrupt diminution in or disappearance of sounds is used as diastolic pressure [44]. This method, still commonly used in the ICU, yields an acceptable value in most situations. Its advantages include low cost, time-honored reliability, and simplicity. Disadvantages include operator variability, susceptibility to environmental noise, and the absence of Korotkoff sounds when pressures are very low. Auscultatory pressures also correlate poorly with directly measured pressures at the extremes of pressure [40, 46]. Therefore, auscultatory pressures must be interpreted with full knowledge of their limitations in the critically ill.
Manual Oscillation Method
The oscillation method has served as the basis for the development of several automated blood pressure monitoring devices, but also continues to be used in manual blood pressure measurement. The first discontinuity in the needle movement of an aneroid manometer indicates the presence of blood flow in the distal artery and is taken as systolic pressure [40]. This oscillation is caused by vibration of the walls of the artery when blood begins to flow through it. The advantages of the oscillation method are its low cost and simplicity. The disadvantages include the inability to measure diastolic pressure, poor correlation with directly measured pressures [40], and lack of utility in situations in which Riva-Rocci measurements are also unobtainable. Aneroid manometers may also be inaccurate: in one study, 34% of all aneroid manometers in use in one large medical system gave inaccurate measurements, even when more lenient standards were used than those advocated by the National Bureau of Standards and the Association for the Advancement of Medical Instrumentation [47]. In the same survey, 36% of the devices were found to be mechanically defective, pointing out the need for regular maintenance. Although the manometers themselves can also be used for auscultatory measurements, oscillometric readings probably provide no advantage over auscultation in the ICU.
Palpation, Doppler, and Pulse-Oximetric Methods
Systolic pressures can be measured by any method that detects flow in a distal artery as the blood pressure cuff is slowly deflated. Palpation of the radial artery is the most commonly used technique; it is most useful in emergency situations in which Korotkoff sounds cannot be heard and an arterial line is not in place. The inability to measure diastolic pressure makes the palpation method less valuable for ongoing monitoring. In addition, palpation obtains no better correlation with direct measurements than the previously described techniques. In one study, variation from simultaneously obtained direct pressure measurements was as high as 60 mm Hg [46]. Like other indirect methods, palpation tends to underestimate actual values to greater degrees at higher levels of arterial pressure. Any method that detects blood flow distal to a sphygmomanometer cuff may
be used in this fashion. Doppler machines are commonly used and may be particularly useful in situations where the pulse is not palpable or environmental noise precludes auscultation [48, 49]. Pulse oximeters have been similarly used and correlate well with other methods; the point at which a plethysmographic trace appears is taken as the systolic pressure [50, 51].
be used in this fashion. Doppler machines are commonly used and may be particularly useful in situations where the pulse is not palpable or environmental noise precludes auscultation [48, 49]. Pulse oximeters have been similarly used and correlate well with other methods; the point at which a plethysmographic trace appears is taken as the systolic pressure [50, 51].
Automated Methods
Automated indirect blood pressure devices measure the arterial blood pressure without manual inflation and deflation of the sphygmomanometer cuff. They operate on one of several principles: Doppler flow, infrasound, oscillometry, volume clamp, arterial tonometry, and pulse wave arrival time.
Doppler Flow
Systems that operate on the Doppler principle take advantage of the change in frequency of an echo signal when there is movement between two objects. Doppler devices emit brief pulses of sound at a high frequency that are reflected back to the transducer [52]. The compressed artery exhibits a large amount of wall motion when flow first appears in the vessel distal to the inflated cuff. This causes a change in frequency of the echo signal, known as a Doppler shift. The first appearance of flow in the distal artery represents systolic pressure. In an uncompressed artery, the small amount of motion does not cause a change in frequency of the reflected signal. Therefore, the disappearance of the Doppler shift in the echo signal represents diastolic pressure [53, 54].
Infrasound
Oscillometry
Oscillometric devices operate on the same principle as manual oscillometric measurements. The cuff senses pressure fluctuations caused by vessel wall oscillations in the presence of pulsatile blood flow [42, 56]. Maximum oscillation is seen at mean pressure, whereas wall movement greatly decreases below diastolic pressure [57]. As with the other automated methods described, the signals produced by the system are processed electronically and displayed in numeric form.
Volume Clamp Technique
The volume clamp method avoids the use of an arm cuff. A finger cuff is applied to the proximal or middle phalanx to keep the artery at a constant size [58]. The pressure in the cuff is changed as necessary by a servocontrol unit strapped to the wrist. The feedback in this system is provided by a photoplethysmograph, which estimates arterial size. The pressure needed to keep the artery at its unloaded volume can be used to estimate the intraarterial pressure [59].
Arterial Tonometry
Arterial tonometry provides continuous noninvasive measurement of arterial pressure, including pressure waveforms. It slightly compresses the superficial wall of an artery (usually the radial). Pressure tracings obtained in this manner are similar to intraarterial tracings. A generalized transfer function can convert these tracings to an estimate of aortic pressure [60]. This method has not yet achieved widespread clinical use. One available system studied in ICU patients had approximately one-third of mean arterial pressure readings which differed by 10 mm Hg or more compared with intraarterial pressure measurements and was associated with significant drift during the course of the study [61]. Another recent system reported more accurate readings in patients undergoing anesthesia [62].
Pulse Wave Arrival Time
The time interval between the R wave of the ECG and the peripheral arrival of the plethysmographic pulse wave may reflect blood pressure change. This theory was tested in 15 critically ill infants and children. The relationship between systolic blood pressure and 1/pulse wave arrival time was not found to be close enough to be clinically useful [63]. However, researchers are actively investigating this area, have found good correlations between refinements of this method and standard measures of blood pressure, and are pursuing improvements of these systems [64, 65]. At present, these systems are not routinely used clinically.
Utility of Noninvasive Blood Pressure Measurements
Only four of the methods described previously (infrasound, oscillometry, Doppler flow, and volume clamp) are associated with significant clinical experience. Of these, methods that use infrasound technology correlate least well with direct measures, with correlation coefficients of 0.58 to 0.84 compared with arterial lines [41, 42, 66]. Therefore, infrasound is rarely used in systems designed for critical care.
Although they have not been consistently accurate, automated methods have the potential to yield pressures as accurate as values derived by auscultation [67, 68 and 69]. Commonly used oscillometric methods can correlate to within 1 mm Hg of the directly measured group average values [42, 70, 71] but may vary substantially from intraarterial pressures in individual subjects, particularly at the extremes of pressure. One study revealed as good a correlation with directly measured pressures as Riva-Rocci pressures have traditionally obtained [42]. Another study demonstrated that mean arterial pressures determined by auscultation were extremely close to those measured by automated devices [68].
When volume clamp methods using a finger cuff have been compared with standard methods in multiple studies [44, 72 73, 74, 75, 76 and 77], these devices have been found to respond rapidly to changes in blood pressure and give excellent correlation in group averages [78]. In one study looking at a large number of measurements, 95% of all measurements using this method were within 10 mm Hg of the directly measured values [67]. Studies by Aitken et al. [74] and Hirschl et al. [44] demonstrated acceptable correlation of volume clamp technique with systolic pressures measured directly. However, other studies have shown clinically significant differences between the volume clamp technique and invasively measured pressures in patients undergoing anesthesia [79, 80 and 81].
One of the proposed advantages of automated noninvasive monitoring is patient safety [82]. Avoiding arterial lines
eliminates the risk of vessel occlusion, hemorrhage, and infection. Automated methods have complications of their own, however. Ulnar nerve palsies have been reported with frequent inflation and deflation of a cuff [83]. Decreased venous return from the limb and eventually reduced perfusion to that extremity can also be seen when the cuff is set to inflate and deflate every minute [82, 83 and 84].
eliminates the risk of vessel occlusion, hemorrhage, and infection. Automated methods have complications of their own, however. Ulnar nerve palsies have been reported with frequent inflation and deflation of a cuff [83]. Decreased venous return from the limb and eventually reduced perfusion to that extremity can also be seen when the cuff is set to inflate and deflate every minute [82, 83 and 84].
In summary, automated noninvasive blood pressure monitoring forms a major component of modern critical care monitoring. Oscillometric and Doppler-based devices are adequate for frequent blood pressure checks in patients without hemodynamic instability, in patient-transport situations in which arterial lines cannot be easily used, and in the severely burned patient, in whom direct arterial pressure measurement may lead to an unacceptably high risk of infection [85]. Automated noninvasive blood pressure monitors have a role in following trends of pressure change [86] and when group averages, not individual measurements, are most important. In general, they have significant limitations in patients with rapidly fluctuating blood pressures and may diverge substantially from directly measured intraarterial pressures. Given these limitations, critical care practitioners should be wary of relying solely on these measurements in patients with rapidly changing hemodynamics or in whom very exact measurements of blood pressure are important.
Direct Invasive Blood Pressure Measurement
Direct blood pressure measurement is performed with an intraarterial catheter. Chapter 3 reviews insertion and maintenance of arterial catheters. Here we discuss the advantages and disadvantages of invasive monitoring compared with noninvasive means.
Arterial catheters contain a fluid column that transmits the pressure back through the tubing to a transducer. A low-compliance diaphragm in the transducer creates a reproducible volume change in response to the applied pressure change. The volume change alters the resistance of a Wheatstone bridge and is thus converted into an electrical signal. Most systems display the pressure in both wave and numeric forms.
Problems in Direct Pressure Monitoring
System-Related Problems
Several technical problems can affect the measurement of arterial pressure with the arterial line. Transducers must be calibrated to zero at the level of the heart. Improper zeroing can lead to erroneous interpretation of essentially accurate measurements. Thrombus formation at the catheter tip can occlude the catheter, making accurate measurement impossible. This problem can be largely eliminated by using a 20-gauge polyurethane catheter, rather than a smaller one, with a slow, continuous heparin flush [87, 88], although this may be associated with heparin-induced thrombocytopenia [89]. Because movement may interrupt the column of fluid and prevent accurate measurement, the patient’s limb should be immobile during readings.
Direct pressure values are also affected by several factors specific to the measurement system itself. An effective design must take into account the transducer system’s natural frequency, which is the frequency at which the system oscillates independent of changes in the measured variable. Signals with wavelengths that approach the natural frequency are amplified and may result in an exaggerated reported pressure [90]. This phenomenon is referred to as overshoot. Above the natural frequency, the signal is attenuated, which can cause the system to report an erroneously low pressure. Accurate recording systems require a natural frequency at least 5 times greater than the fundamental frequency (i.e., the range of expected heart rates) [91, 92]. This is necessary to account for the multiple harmonic frequencies produced along with the main (fundamental) frequency.
The frequency response of the system is a phenomenon not only of transducer design but of the tubing and the fluid in it. The length, width, and compliance of the tubing all affect the system’s response to change. Designs should use tubing shorter than 60 cm [93]. Small-bore catheters are preferable because they minimize the mass of fluid that can oscillate and amplify the pressure [92]. The compliance of the system (the change in volume of the tubing and the transducer for a given change in pressure) should be low [92]. In addition, bubbles in the tubing can affect measurements in two ways. Large amounts of air in the measurement system damp the system response and cause the system to underestimate the pressure [94]. This is usually easily detectable. Small air bubbles cause an increase in the compliance of the system and can significantly amplify the reported pressure [92, 93 and 94].
Site-Related Problems
Other problems arise in relation to the location of catheter placement. The radial artery is the most common site of arterial cannulation for pressure measurement. This site is accessible and can be easily immobilized to protect both the catheter and the patient. The major alternative site is the femoral artery. Both sites are relatively safe for insertion [87, 95, 96]. A systematic review of 19,617 radial, 3,899 femoral, and 1,989 axillary cannulations found that serious complications occurred infrequently (less than 1% of cannulations) and were similar between the sites [97]. In particular, and somewhat surprisingly, rates of infection appear similar between the femoral and radial sites [97, 98]. The ulnar, brachial, dorsalis pedis, and axillary arteries are also used with some frequency [96, 97, 99, 100]. Although there are a number of theoretical considerations about comparing blood pressures from one site to another, there are little data for critically ill patients. In 14 septic surgical patients on vasopressors, radial pressures were significantly lower than femoral arterial pressures. In 11 of the 14, vasopressor dose was reduced based on the femoral pressure without untoward consequences; after vasopressors were discontinued, radial and femoral pressures equalized. The authors concluded that clinical management based on radial artery pressures may lead to excessive vasopressor administration [101]. Similar significant differences in systolic pressures between the radial and femoral sites were found in the reperfusion phase of liver transplantation, although mean arterial pressures did not differ [102]. However, another larger observational study in critically ill patients [103], and one in hypotense anesthetized patients [104], found no clinically meaningful differences in blood pressures between the sites. Although data are sparse, mean arterial pressure readings between the radial and femoral sites are probably interchangeable.
Advantages
Despite technical problems, direct arterial pressure measurement offers several advantages. Arterial lines actually measure the end-on pressure propagated by the arterial pulse. In contrast, indirect methods report the external pressure necessary
either to obstruct flow or to maintain a constant transmural vessel pressure. Arterial lines can also detect pressures at which Korotkoff sounds are either absent or inaccurate. Arterial lines provide a continuous measurement, with heartbeat-to-heartbeat blood pressures. In situations in which frequent blood drawing is necessary, indwelling arterial lines eliminate the need for multiple percutaneous punctures. Finally, analysis of the respiratory change in systolic or pulse pressure [105] may provide important information on cardiac preload and fluid responsiveness.
either to obstruct flow or to maintain a constant transmural vessel pressure. Arterial lines can also detect pressures at which Korotkoff sounds are either absent or inaccurate. Arterial lines provide a continuous measurement, with heartbeat-to-heartbeat blood pressures. In situations in which frequent blood drawing is necessary, indwelling arterial lines eliminate the need for multiple percutaneous punctures. Finally, analysis of the respiratory change in systolic or pulse pressure [105] may provide important information on cardiac preload and fluid responsiveness.
Conclusions
Indirect methods of measuring the blood pressure estimate the arterial pressure by reporting the external pressure necessary to either obstruct flow or maintain a constant transmural vessel pressure. Arterial lines measure the end-on pressure propagated by the arterial pulse. Direct arterial pressure measurement offers several advantages. Although an invasive line is required, the reported risk of complications is low [97]. Arterial lines provide a heartbeat-to-heartbeat measurement, can detect pressures at which Korotkoff sounds are either absent or inaccurate, and do not require repeated inflation and deflation of a cuff. Additionally, they provide easy access for phlebotomy and blood gas sampling, and they may provide additional information about cardiac status. Regardless of the method used, the mean arterial pressure should generally be the value used for decision-making in most critically ill patients.
Electrocardiographic Monitoring
Almost all ICUs in the United States routinely perform continuous ECG monitoring. Continuous ECG monitoring combines the principles of ECG, which have been known since 1903, with the principles of biotelemetry, which were first put into practical application in 1921 [106, 107]. Here we review the principles of arrhythmia monitoring, automated arrhythmia detection, and the role of automated ST segment analysis.
ECG monitoring in most ICUs is done over hard-wired apparatus. Skin electrodes detect cardiac impulses and transform them into an electrical signal, which is transmitted through wires directly to the signal converter and display unit. This removes the problems of interference and frequency restrictions seen in telemetry systems. Although this comes at the cost of reduced patient mobility, mobility is often not an immediate concern for this group of patients.
Arrhythmia Monitoring in the Intensive Care Unit
The American Heart Association’s Practice Standards guideline considers continuous ECG monitoring a class I intervention for all patients with indications for intensive care, regardless of whether the patient’s primary admitting diagnosis related to a cardiac problem [108]. Approximately 20% of ICU patients in a general ICU have significant arrhythmias, mostly atrial fibrillation or ventricular tachycardia [109], with a circadian variation in arrhythmia onset, peaking at about 2 PM for ventricular fibrillation [110]. There is also a substantial incidence of arrhythmia following major surgery [111, 112 and 113]. Although no studies address whether monitoring for arrhythmias in a general ICU population alters outcomes, this monitoring is generally accepted and considered standard care [108]. In postmyocardial infarction patients, on the other hand, the data are compelling. Arrhythmia monitoring was shown to improve the prognosis of patients admitted to the ICU for acute myocardial infarction many years ago [114, 115 and 116]. It has been a standard of care in the United States since that time. Although ventricular tachycardia and fibrillation after myocardial infarction have declined in frequency over the years, they still occur in about 7.5% of patients [117]. Monitoring enables the rapid detection of these potentially lethal rhythms. Because thrombolytic therapy for acute myocardial infarction also leads to a slight increase in arrhythmias within 8 to 12 hours after successful reperfusion [118, 119 and 120], the incidence of arrhythmias can be used as one indicator of successful reperfusion.
Evolution of Arrhythmia Monitoring Systems for Clinical Use
After ICUs implemented continuous ECG monitoring, practitioners recognized some deficiencies with the systems. Initially, the responsibility for arrhythmia detection was assigned to specially trained coronary care nurses. Despite this, several studies documented that manual methods failed to identify arrhythmias, including salvos of ventricular tachycardia, in up to 77% of cases [121, 122 and 123]. This failure was probably due to an inadequate number of staff nurses to watch the monitors, inadequate staff education, and faulty monitors [122]. Subsequently, monitors equipped with built-in rate alarms that sounded when a preset maximum or minimum rate was detected proved inadequate because some runs of ventricular tachycardia are too brief to exceed the rate limit for a given time interval [121, 123]. Ultimately, computerized arrhythmia detection systems were incorporated into the monitors. The software in these systems is capable of diagnosing arrhythmias based on recognition of heart rate, variability, rhythm, intervals, segment lengths, QRS complex width, and morphology [124, 125]. These systems have been validated in coronary care and general medical ICUs [123, 126]. Computerized arrhythmia detection systems are well accepted by nursing personnel, who must work most closely with them [127].
Ischemia Monitoring
Just as simple monitoring systems can miss episodes of ventricular tachycardia and ventricular fibrillation, they can fail to detect significant episodes of myocardial ischemia [128]. This is either because the episode is asymptomatic or because the patient’s ability to communicate is impaired by intubation or altered mental status. ECG monitoring systems with automated ST segment analysis have been devised to attempt to deal with this problem.
In most ST segment monitoring systems, the computer initially creates a template of the patient’s normal QRS complexes. It then recognizes the QRS complexes and the J points of subsequent beats and compares an isoelectric point just before the QRS with a portion of the ST segment 60 to 80 msec after the J point [129]. It compares this relationship to that of the same points in the QRS complex template. The system must decide whether the QRS complex in question was generated and conducted in standard fashion or whether the beats are aberrant, which negates the validity of comparison. Therefore, an arrhythmia detection system must be included in all ischemia
monitoring systems designed for use in the ICU. Standard systems can monitor three leads simultaneously. These leads are usually chosen to represent the three major axes (anteroposterior, left-right, and craniocaudal). The machine can either display these axes individually or sum up the ST segment deviations and display them in a graph over time [129].
monitoring systems designed for use in the ICU. Standard systems can monitor three leads simultaneously. These leads are usually chosen to represent the three major axes (anteroposterior, left-right, and craniocaudal). The machine can either display these axes individually or sum up the ST segment deviations and display them in a graph over time [129].
Automated ST segment analysis has gained widespread popularity among cardiologists. Since 1989, the American Heart Association has recommended that ischemia monitoring should be included in new monitoring systems developed for use in the coronary care unit [130]. In patients admitted for suspected acute coronary syndromes, ischemia is frequently both silent and strongly associated with adverse events after discharge [108, 131]. While noting that no randomized clinical trials document improved patient outcomes when automated ST segment monitoring is used to detect ischemia, the American Heart Association recommends ST segment monitoring for patients with a number of primary cardiac issues (e.g., acute coronary syndromes), based on expert opinion. The guidelines make no statement regarding ST segment monitoring for ICU patients [108].
Newer Techniques
Because conventional 3-lead monitoring detects only about one-third of transient ischemic events in patients with unstable coronary syndromes [132], some authors now advocate the use of continuous 12-lead ECG systems in the care of acute coronary syndromes [133, 134]. Although 12-lead monitoring detects additional arrhythmias, studies have not compared outcomes between standard and 12-lead monitoring approaches in ICU patients. Other proposed enhancements to continuous ECG monitoring include signal-averaged ECG, QT dispersion, QT interval beat-to-beat variability, and heart rate variability [135]. Although associated with subsequent arrhythmic events, these have not yet reached common clinical use.
Technical Considerations
As with any other biomedical measurement, technical problems can arise when monitoring cardiac rhythms. Standards have been devised to guide manufacturers and purchasers of ECG monitoring systems [130, 136].
The possibility of electrical shock exists whenever a patient is directly connected to an electrically operated piece of equipment by a low-resistance path. Electrical shocks would most commonly occur with improper grounding of equipment when a device such as a pacemaker is in place. Necessary precautions to avoid this potential catastrophe include (a) periodic checks to ensure that all equipment in contact with the patient is at the same ground potential as the power ground line, (b) insulating exposed lead connections, and (c) using appropriately grounded plugs [137, 138].
The size of the ECG signal is important for accurate recognition of cardiac rate and rhythm. Several factors may affect signal size. The amplitude can be affected by mismatching between skin-electrode and preamplifier impedance. The combination of high skin-electrode impedance, usually the result of poor contact between the skin and electrode, with low-input impedance of the preamplifier can decrease the size of the ECG signal. Good skin preparation, site selection, and conducting gels can promote low skin-electrode impedance. A high preamplifier input impedance or the use of buffer amplifiers can also improve impedance matching and thereby improve the signal obtained. Another factor that affects complex size is critical damping, the system’s ability to respond to changes in the input signal. An underdamped system responds to changes in input with displays that exaggerate the signal, called overshoot. An overdamped system responds slowly to a given change and may underestimate actual amplitude. The ECG signal can also be affected by the presence of inherent, unwanted voltages at the point of input. These include the common mode signal, a response to surrounding electromagnetic forces; the direct current skin potential produced by contact between the skin and the electrode; and a potential caused by internal body resistance. Finally, the ECG system must have a frequency response that is accurate for the signals being monitored. Modern, commercially available systems have incorporated features to deal with each of these problems.
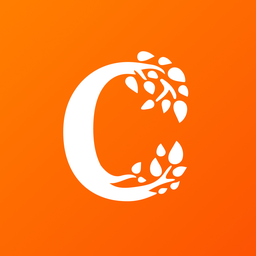
Full access? Get Clinical Tree
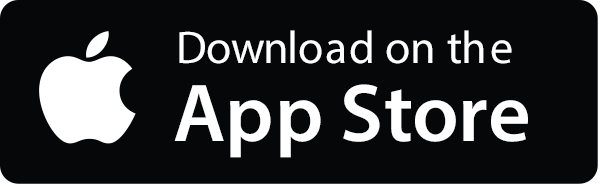
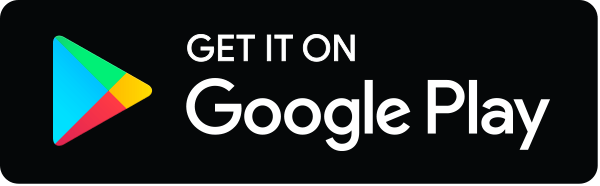