Chapter Outline
Detectors of Infrared Radiation
Infrared Wavelength and Anesthetic Agent Specificity
Sampling Systems and Infrared Analysis
Infrared Photoacoustic Spectrometer
WATER VAPOR AND ACCURACY OF CAPNOMETERS
COLORIMETRIC CARBON DIOXIDE DETECTORS
APPLICATIONS OF GAS MONITORING
COMPLICATIONS OF GAS MONITORING
CREDENTIALING FOR THE USE OF GAS MONITORING
AMERICAN SOCIETY OF ANESTHESIOLOGISTS STANDARDS FOR POSTANESTHESIA CARE
Overview
The gases of interest to the anesthesia caregiver include oxygen, carbon dioxide, nitrous oxide, and the potent inhaled anesthetic agents. Other gases that may be relevant in certain situations are nitrogen, helium, nitric oxide, and xenon. Although gas monitors from different manufacturers may appear to offer various options to the user, ultimately, these monitors use one or more of a limited number of technologies to make the analysis and present the data.
∗ Parts of this chapter are reproduced by permission from Eisenkraft JB: Respiratory gas monitoring. In Reich DL et al, editors: Monitoring in Anesthesia and Perioperative Care , New York, 2011, Cambridge University Press.
The monitoring of respired gases has evolved considerably over the past few years. Contemporary systems are reliable, accurate, have rapid response times, and are becoming less expensive as a result of competition among manufacturers. Early gas monitoring systems were large, stand-alone units that usually were placed on a shelf on the anesthesia machine ( Fig. 8-1 ). As a result of advances in technology and miniaturization, on many contemporary anesthesia workstations, gas analysis is performed in one component module of a modular physiologic monitoring system ( Fig. 8-2 ). This chapter is intended to provide a framework for understanding the methods by which respiratory gases are analyzed as well as clinical applications, limitations, and pertinent standards of care.


Gas Sampling Systems
For a respired gas mixture to be analyzed, either the gas must be brought to the analyzer, or the analyzer must be brought to the gas in the airway. A fuel cell oxygen analyzer located in the breathing system by the inspiratory unidirectional valve is one example of bringing the analyzer to the gas in the circuit. Figure 8-3 shows two mainstream analyzer modules. Because gas is not removed from the circuit for analysis elsewhere, this is termed a nondiverting or mainstream analyzer. Alternatively, and more commonly, the gas to be analyzed is continuously sampled from the vicinity of the patient’s airway and is conducted via fine-bore tubing to the analyzer unit ( Fig. 8-4 ). This arrangement is termed a sidestream or diverting system because the gas is diverted from the airway for analysis elsewhere.


Gas analysis is performed in real time so that changes can be rapidly appreciated by the anesthesia caregiver. It is therefore important to know the total response time of the complete system. The total response time is composed of two components: the transit time and the rise time. The transit time is the time lag for the gas sample to reach the analyzer; this term applies only to systems that use diverting gas sampling. The rise time is the time taken by the analyzer to react to the change in gas concentration. An analyzer’s reponse to a sudden (square wave) change in gas concentration is generally sigmoid in shape, so that rise time is specified as the time to change from 10% to 90% of the sudden total change in gas concentration at the analyzer inlet ( Fig. 8-5 ). As an example, the Datex Compact Airway module (GE Healthcare, Waukesha, WI; see Fig. 8-1, right ) with a 3-meter sampling line and 200 ± 20 mL/min gas sampling rate typically has a sampling delay time of 2.5 seconds and a total response time of 2.9 seconds, which includes the sampling delay and rise time. For comparison, the Capnostat 5 mainstream carbon dioxide sensor (Philips Respironics, Wallingford, CT) is specified to have a rise time of less than 60 ms. A rapid total system response time is essential for accurate concentration readings and high-fidelity waveforms.

Nondiverting (Mainstream) Systems
In nondiverting systems, gas flows past the analyzer interface placed in the main gas stream. Until recently, mainstream analysis at the patient’s airway was possible only for carbon dioxide using infrared (IR) technology (Mainstream, Philips Respironics) and by the inspiratory unidirectional valve for oxygen using a fuel cell. As a result of advances in miniaturization, mainstream multigas analysis at the patient’s airway is now available (Phasein, Danderyd, Sweden); this permits rapid acquisition of breath-by-breath data for carbon dioxide, nitrous oxide, oxygen, and the five potent inhaled anesthetics. Although mainstream analyzers overcome the gas sampling problem, they require a special airway adapter and analysis module to be placed in the breathing system near the patient’s airway. From this location, the analyzers produce a sharp concentration-versus-time waveform in real time, but they may be vulnerable to damage with repeated drops onto hard surfaces. New designs are lightweight, such as the Phasein IRMA sensor head, which uses a miniaturized, spinning filter wheel that weighs only 1 oz. Such designs add only a small amount of dead space, and some, such as the Capnostat 5, use solid-state technology. In addition, waste gas scavenging is not necessary with nondiverting systems.
Mainstream analyzer modules are subject to interference by water vapor, secretions, and blood. Because condensed water blocks all IR wavelengths, leaving too low a source intensity to make a measurement, spurious carbon dioxide readings might result. The cuvette’s window may be heated (usually to 41° C), or it may be coated with water-repellant material to prevent such condensation and interference. Adding the mainstream analyzer to the breathing system creates two additional interfaces for a potential breathing circuit disconnection. Disposable breathing system adapters are now available, whereas previously, the nondisposable adapters required cleaning between patient uses. In addition, a mainstream carbon dioxide analyzer is now available for patients receiving oxygen via nasal cannula (Cap-ONE, Nihon Kohden America, Foothill Ranch, CA).
Sidestream (Diverting) Systems
Compared with mainstream analyzers, the advantages of diverting analyzers are that, because they are remote from the patient, they can be of any size and therefore offer more versatility in terms of monitoring capabilities. They can be used when the monitor must be remote from the patient, such as in magnetic resonance imaging (MRI) or radiation therapy. The sampled gas is continually drawn from the breathing circuit via an adapter placed between the circuit and the patient’s airway (the Y-piece in a circle breathing system); it passes through a filter or water trap ( Fig. 8-1 , arrows ) before entering the analyzer. The gas sampling flow rate is usually about 200 mL/min, with a range of 50 to 250 mL/min. Disadvantages include problems with the catheter sampling system, such as clogging with secretions or water, kinking, failure of the sampling pump, slower total system response time (although usually <3 seconds), and artifacts when the gas sampling rate is poorly matched to the patient’s inspiratory and expiratory gas flow rates.
Rapid respiratory rates and long sampling catheter lines may decrease the accuracy of the readings and the fidelity of the tracings. This is because there would be samples from many breaths stored in the catheter, and the breaths could “smudge” into one another, thereby “dampening” the tracing with loss of clear peaks and troughs. If a diverting system is used with a very small patient, such as a neonate, and the gas sampling rate exceeds the patient’s expiratory gas flow rate, spurious readings may result because the expired gas will be contaminated by fresh gas. Similarly, if an uncuffed tracheal tube is used and a leak develops between the tube and the trachea, the gas sampling pump may draw room air into the tracheal tube and into the analyzer.
Ideally, the gas sampling flow rate should be appropriate for the patient and for the breathing circuit used. Thus the sampling flow rate may limit the use of low-flow or closed-circuit anesthesia techniques. If the gas sampling rate exceeds the fresh gas inflow rate, the potential exists for negative pressures to be created in the breathing system. Once the sampled gas has been analyzed, it should be directed to the waste gas scavenging system or returned to the patient’s breathing system.
Leaks in the gas sampling line, both inside the monitor and between the patient’s airway and the monitor inlet, will result in erroneous readings that may or may not be obvious. These monitors require calibration using a certified standard gas mixture that is directed into the monitor’s gas inlet connection. A leak inside the monitor that allows room air to contaminate the gas sample would result in miscalibration.
In multigas analyzers that incorporate a paramagnetic oxygen sensor, simultaneous room air sampling (10 mL/min) is required to provide a reference. This air is therefore added to the waste gas exiting the monitor at a rate of 10 mL/min; it may then be returned to the patient circuit. This might create a problem during closed-circuit anesthesia because nitrogen, albeit at a rate of about 8 mL/min, would be added to the breathing circuit (see Paramagnetic Oxygen Analyzers later in this chapter).
Units of Measurement
The respiratory tract and the anesthesia delivery system contain respired gases in the form of molecules that are in constant motion. When the molecules strike the walls of their container, they give rise to pressure, defined as force per unit of area; the greater the number of gas molecules present, the greater the pressure exerted for any given temperature. Dalton’s law of partial pressures states that the total pressure exerted by a mixture of gases is equal to the arithmetic sum of the partial pressures exerted by each gas in the mixture. The total pressure of all gases in the anesthesia system at sea level is equivalent to approximately 760 mm Hg. Although anesthetic gas monitors may display data expressed in millimeters of mercury (mm Hg), kilopascals (1 kPa = 7.5 mm Hg), or as volumes percent (vol%), it is important to understand in principle how the measurement was made. The reader should understand the difference between partial pressure , an absolute term, and volumes percent, an expression of a proportion, or ratio.
If the partial pressure of one component of a gas mixture is known, a reading in volumes percent can be computed as follows:
Partial pressure of gas ( mm Hg ) ÷ Total pressure of all gases ( mm Hg ) × 100 %
Number of Molecules (Partial Pressure)
An analytic method based on quantifying a specific property of a gas molecule determines in absolute terms—that is, in millimeters of mercury or kilopascals—the number of molecules of that gas that are present. Gas molecules composed of two or more dissimilar atoms—such as carbon dioxide, nitrous oxide, and the potent inhaled anesthetics—have bonds between their component atoms. Certain wavelengths of IR radiation excite these molecules, stretching or distorting the bonds, and the molecules also absorb the radiation. Carbon dioxide molecules absorb IR radiation at a wavelength of approximately 4.3 μm. The greater the number of molecules of carbon dioxide present, the more radiation at 4.3 μm absorbed. This property of the carbon dioxide molecule is applied in the IR carbon dioxide analyzer. Because the total amount of IR radiation absorbed at a specific wavelength is determined by the number of molecules present, and the motion of each molecule contributes to the total pressure, the amount of radiation absorbed is a function of partial pressure; thus, an IR analyzer measures partial pressure.
In the analysis of gases by Raman spectroscopy, as was used in the Datex-Ohmeda Rascal II analyzer (GE Healthcare), a helium-neon laser emits monochromatic light at a wavelength of 633 nm. When this light interacts with the intramolecular bonds of specific gas molecules, it is scattered and reemitted at wavelengths different from that of the incident monochromatic light. Each reemission wavelength is characteristic of a specific gas molecule present in the gas mixture and therefore is a function of its partial pressure. Thus Raman spectroscopy also measures partial pressures.
A sufficient number of molecules of any gas to be analyzed—that is, adequate partial pressures—must be present to facilitate gas analysis by the IR and Raman technologies. These systems also must be pressure compensated if analyses are being made at ambient pressures other than those used for the original calibration of the systems.
Measurement of Proportion (Volumes Percent)
Another approach to gas analysis is to separate the molecular component species of a gas mixture and determine what proportion (percentage) each gas contributes to the total (100%). This approach is applied in mass spectrometry. Thus, if 21 molecules of oxygen were in a sample of gas containing 100 molecules, oxygen would represent 21% of the gas sample and therefore might reasonably be assumed to represent 21% of the original gas mixture. The result is expressed as 21 vol% or as a fractional concentration (0.21). This monitor does not measure partial pressures; it measures only proportions. If the system is provided with an absolute pressure reading that is equivalent to 100%, the basic measured proportions can be converted to readings in millimeters of mercury. In the above example, if 100% were made equivalent to 760 mm Hg, oxygen would have a calculated partial pressure of 159 mm Hg (760 × 21%).
These fundamental differences in the approaches to gas analysis and their basic units of measurement are important, particularly when the data presented by these monitors are interpreted in a clinical setting and may affect patient management.
Gas Analysis Technologies
Contemporary respiratory multigas analyzers use some form of IR spectroscopy to measure carbon dioxide, nitrous oxide, and the potent inhaled anesthetic agents. The same multigas analyzers measure oxygen by a paramagnetic, rapid-responding analyzer or by a fuel cell (slow or rapid responding). Although some technologies are no longer in general clinical use, it is worthwhile to review them briefly to appreciate their principles of operation and how monitoring has evolved.
Mass Spectrometry
For many anesthesia caregivers, the term mass spec is used as if it were synonymous with respiratory gas analysis. Indeed, many anesthetic record forms still incorrectly include this term, but this technology is no longer in routine clinical use. It was, however, the first multigas monitoring system in widespread clinical use, following a description by Ozanne and colleagues in 1981.
The mass spectrometer is an instrument that allows the identification and quantification, on a breath-by-breath basis, of up to eight of the gases commonly encountered during the administration of an inhalational anesthetic. These gases include oxygen, nitrogen, nitrous oxide, halothane, enflurane, and isoflurane. Other agents—such as helium, sevoflurane, argon, and desflurane—could sometimes be added or substituted if desired. Although the technology of mass spectrometry had been available for many years, analyzer units dedicated to a single patient were too expensive for routine use in each operating room (OR). In 1981, the concept of a shared, or multiplexed, system was introduced. This arrangement allowed one centrally located analyzer to function as part of a computerized, multiplexed system that could serve up to 31 patient sampling locations (ORs and recovery room or intensive care unit [ICU] beds) on a time-share basis. The two multiplexed systems that became widely used were the Perkin Elmer system, which later became the Marquette Advantage system, and the System for Anesthetic and Respiratory Analysis (SARA).
Principles of Operation
The mass spectrometer analyzer unit separates the components of a stream of charged particles (ions) into a spectrum according to their mass/charge (m/z) ratios. The relative abundance of ions at certain specific m/z ratios is determined and is related to the fractional composition of the original gas mixture. The creation and manipulation of ions is carried out in a high vacuum (10 −5 mm Hg) to avoid interference by outside air and to minimize random collisions among the ions and residual gases.
The most common design of mass spectrometer was the magnetic sector analyzer, so called because it uses a permanent magnet to separate the ion beam into its component ion spectra ( Fig. 8-6 ). A stream of gas (250 mL/min) to be analyzed is continuously drawn by a sampling pump from an airway connector via a long nylon catheter, an example of a sidestream-sampling system. During transit through the sampling catheter, the pressure decreases from atmospheric pressure, usually 760 mm Hg, in the patient circuit to approximately 40 mm Hg by the inlet of the analyzer unit. A very small amount of the gas actually sampled from the circuit, approximately 10 −6 mL/sec, enters the analyzer unit’s high-vacuum chamber through the molecular inlet leak. The gas molecules are then bombarded by an electron beam, which causes some of the molecules to lose one or more electrons and become positively charged ions. Thus an oxygen molecule (O 2 ) might lose one electron and become an oxygen ion (O 2 + ) with one positive charge. The m/z ratio would therefore be 32/1, or 32. If the oxygen molecule lost two electrons, it would gain two positive charges, and the resulting ion (O 2 2+ ) would have an m/z ratio of 32/2, or 16. The process of electron bombardment also causes large molecules—such as halothane, enflurane, and isoflurane—to become fragmented, or “cracked,” into smaller, positively charged ions.

The positive ions created in the analyzer are then focused into a beam by the electrostatic fields in the ion source, directed through a slit to define an exact shape for the beam, and accelerated and directed into the field of the permanent magnet. The magnetic field influences the direction of the ions and causes each ion species to curve in a trajectory whose arc is related to its m/z ratio. The effect is to create several separate ion beams exiting the magnetic field. The separated beams are directed to individual collectors, which detect the ion current and transmit it to amplifiers that create output voltages in relation to the abundance of the ion species detected. The collector plates are positioned so that an ion with a specific m/z ratio strikes a specific collector. The heaviest ions are deflected the least and travel the furthest before striking a collector (see Fig. 8-6 ). Collectors for these heavy ions are therefore located farthest from the ion source. Summing and other computer software measure the total voltage from all the collector circuits as well as measuring the individual voltages from each collector. Total voltage is considered equivalent to 100% of the analyzed gas mixture.
Individual gas collector circuit voltages are expressed as percentages of the total voltage and are displayed as percentages of the sampled gas mixture. Thus if the voltage from the oxygen collector circuit (m/z ratio = 32) represented 30% of the total voltage from all the collector circuits, oxygen would be read as constituting 30% of the total gas mixture analyzed. The Marquette Advantage and SARA systems used magnetic sector analyzers that had up to eight collectors and therefore were able to detect and analyze up to eight different ion types and their parent gases.
The mass spectrometer functions as a proportioning system for the components of a gas mixture. When it displays each of the components of the mixture as a percentage of the total, it makes the assumption that all the gases present have been detected. If ambient (atmospheric) pressure information is entered into the software, the measured percentages or proportions can be converted to readings in millimeters of mercury (i.e., partial pressures). It must be remembered that the mass spectrometer does not measure partial pressures; it calculates them from the measured proportions and the atmospheric pressure information that must be supplied to it. Thus
Partial pressure ( mm Hg ) = Fractional concentration × Total pressure ( mm Hg )
Usually, because the mass spectrometer was sampling respired gases from the patient circuit, the total pressure entered into the computer was ambient pressure minus 47 mm Hg, the latter representing the saturated vapor pressure of water at body temperature (37° C). Thus at sea level (760 mm Hg), a pressure of 713 mm Hg would be entered into the mass spectrometer software to be apportioned among the gases present. The mass spectrometer readings, when displayed in millimeters of mercury, represent somewhat of a compromise because inspired gas usually is not fully saturated with water vapor, whereas expired gas usually is. If an incorrect value for total ambient pressure were to be entered into the mass spectrometer software, all readings in millimeters of mercury would be incorrect, but the readings in volumes percent would be correct. For example, assume that end-tidal carbon dioxide is measured as 5% by the mass spectrometer and that ambient pressure is 760 mm Hg. The reading in millimeters of mercury will be 35.65 ([760 − 47] × 5%). If a value of 500 mm Hg is entered instead of 713 mm Hg, the reading will be 25 mm Hg (500 × 5%). In any case of doubt, the astute clinician would revert to the reading in volumes percent; hence the importance of understanding how the displayed value is obtained.
Shared Mass Spectrometry Systems
Multiplexing permitted the sharing of one (rather expensive) mass spectrometer analyzer unit among up to 31 sampling locations, or stations. In a shared system, the gas from each sampling location is directed in sequence by the multiplexing valve system to the mass spectrometer for analysis. In a multiplexed system, the time between analyses at any particular location therefore depended on 1) the number of breaths analyzed from each sampling location (i.e., dwell time), 2) the number of sampling locations in use, 3) the priority settings, and 4) in the case of “stat” samples, the distance between sampling locations and the analyzer, which was up to 300 feet in some installations.
Use of multiplexed mass spectrometry systems led anesthesia caregivers to appreciate the value of continual (i.e., frequently repeated), although not continuous (without interruption), respiratory gas monitoring and the limitations—the main one being that the systems were shared, which meant that data were updated only intermittently.
In October 1986, the American Society of Anesthesiologists (ASA) first approved standards for basic intraoperative monitoring. As the standards evolved, the requirement for continuous capnometry was not met by a shared mass spectrometry system. The systems were expensive to install and maintain, and they were not always easily upgradable when the new agents desflurane and sevoflurane were introduced. In addition, the long sampling catheters combined with rapid respiratory rates led to significant artifact; the sampling pump could cause considerable negative pressure in the breathing system, and when the system failed, all the monitored sites were affected. Practitioners demanded continuous gas monitoring. The most important functions of a multiplexed mass spectrometry system could be served by other dedicated gas monitors, and the multiplexed, mass spectrometer–based systems became extinct.
Dedicated (Stand-Alone) Mass Spectrometry Systems
A number of smaller stand-alone mass spectrometers were developed for dedicated single-patient use. One model, the Ohmeda 6000 Multigas Analyzer, was a quadrupole-filter mass spectrometer. It worked on the principle that, with regard to the m/z ratio of ions, a controlled electrostatic field can prevent all but a narrow range of charged particles from reaching a target. In the Ohmeda 6000 unit, the gas sampling rate was fixed at 30 mL/min. One advantage of the quadrupole system was that it could be adapted to measure new or additional agents by changes in software only. A number of other stand-alone, quadrupole-filter mass spectrometers were marketed, but their production was discontinued as a result of cost and reliability issues.
Infrared Analysis
The IR spectrum ranges between wavelengths of 0.40 μm and 40 μm. Measurement of the energy absorbed from a narrow band of wavelengths of IR radiation as it passes through a gas sample can be used to measure the concentrations of certain gases. Asymmetric, polyatomic, polar molecules—such as carbon dioxide, nitrous oxide, water, and the potent volatile anesthetic agents—absorb IR energy when their atoms rotate or vibrate asymmetrically; this results in a change in dipole moment, the charge distribution within the molecule. The nonpolar molecules argon, nitrogen, helium, xenon, and oxygen do not absorb IR energy. Because the number of gas molecules in the path of the IR energy beam determines the total absorption, IR analyzers measure the partial pressure.
IR analyzers are classified as dispersive or nondispersive. In a dispersive analyzer, after passing through the gas sample, the radiation emitted by an IR source is separated, or dispersed, into the component wavelengths and is arranged sequentially. When gas to be analyzed is put in the path of the spectrum of radiation, it absorbs radiation in one or more parts of the spectrum. By examining the entire spectrum, a plot of absorbance versus wavelength is obtained ( Fig. 8-7 ), from which the gas composition can be analyzed and quantified, provided the gases in the mixture have characteristic absorption peaks.

In the nondispersive analyzer, radiation from the IR source is filtered to allow passage of only the specific wavelength bands, for which the gases of interest have distinct absorption peaks. The gas sample is placed between the filter and the IR detector ( Fig. 8-8 ) or between the IR source and the filter ( Fig. 8-9 ). With the exception of the Andros, the IR analyzers used clinically are predominantly of the nondispersive type.


Carbon dioxide, nitrous oxide, and anesthetic gases exhibit absorption of radiation at unique bands in the IR spectrum (see Fig. 8-7 ). Carbon dioxide molecules absorb strongly between 4.2 and 4.4 μm, whereas nitrous oxide molecules absorb strongly between 4.4 and 4.6 μm and less strongly at 3.9 μm. The potent volatile anesthetic agents have strong absorption bands at 3.3 μm and throughout the range 8 to 12 μm.
The close proximity of the nitrous oxide and carbon dioxide absorption bands may cause some analyzers to be affected by high concentrations of nitrous oxide. The impact of this cross-interference—that is, the overlapping of absorption bands of other gases—can vary significantly among devices. The use of narrow-band sources or narrow-band filters with sufficiently small bandwidths can effectively reduce the impact of cross interference. The presence of other gases, which may or may not have overlapping absorption bands, can also affect the measurement. This phenomenon is called collision broadening or pressure broadening because molecular collisions result in a change in the dipole moment of the gas being analyzed; thus the IR absorption band is broadened, and the apparent absorption at the measurement wavelength may be altered. In a typical IR carbon dioxide analyzer, 95% oxygen causes a 0.5% decline in the measured carbon dioxide. Nitrous oxide causes a more substantial increase of approximately 0.1% carbon dioxide per 10% nitrous oxide because of collision broadening. Contemporary multigas analyzers can automatically compensate for the effect of collision broadening if they measure the concentrations of interfering gases.
A simple nondispersive IR analyzer (see Fig. 8-8 ) consists of the following basic elements:
- 1.
A source of IR radiation, typically a heated black body. A black body radiator is a theoretical object that is totally absorbent to all thermal energy that falls on it; it does not reflect any light and therefore appears black. As it absorbs energy, it heats up and reradiates the energy as electromagnetic radiation. Heating the black body causes emission of IR radiation.
- 2.
A sample cell, or cuvette. The gas to be analyzed is drawn through the cuvette by a sampling pump.
- 3.
A detector that generates an output signal. The signal is related to the intensity of the IR radiation that falls on it.
- 4.
A narrow-band pass filter. This filter allows only radiation at the wavelength bands of interest to pass through; it is interposed between the IR source and the cuvette (see Fig. 8-8 ) or between the cuvette and the detector (see Fig. 8-9 ). The intensity of radiation reaching the detector is inversely related to the concentration of the specific gas being measured.
A number of sources of IR radiation can be used to produce a broad spectrum of IR radiation. Light sources made of tungsten wires or ceramic resistive materials heated to 1500 to 4000 K emit energy over a broad wavelength range that includes the absorption spectrum of the respiratory gases. The radiation may be pulsed electronically (see Fig. 8-9 ) or, if constant, may be made intermittent by being interrupted mechanically, or “chopped,” such as with a filter wheel ( Fig. 8-10 ). Because energy output of IR light sources tends to drift, optical systems have been designed to stabilize the analyzers. Three common designs are distinguished by their use of single or dual IR beams and by their use of positive or negative filtering.

Single-Beam Positive Filter
In single-beam positive-filter designs, the IR beam may be divided in time or in space. If the IR beam is divided in time, precision optical band-pass filters mounted on a chopper wheel spinning at 40 to 250 rpm sequentially interrupt a single IR beam. The beam retains energy at a narrow wavelength band during each interruption. If the IR beam is divided in space, after passing through the cuvette, the beam is divided into separate paths using beam splitters and mirrors before passing through optical filters. For each gas of interest, a pair of band-pass filters is selected at an absorption peak and at a reference wavelength at which relatively little absorption occurs. The chopped IR beam then passes through a cuvette containing the sample gas. The ratio of intensity of the IR beam for each pair of filters is proportional to the partial pressure of the gas and is insensitive to changes in the intensity of the IR source.
Single-Beam Negative Filter
In the single-beam negative-filter design, the filters usually are gas-filled cells mounted in a spinning wheel. During each interruption, the IR beam retains energy at all wavelengths except those absorbed by the gas. The chopped IR beam then passes through a cuvette containing the sample gas. Analogous to the positive-filter design previously described, the ratio of IR beam intensity for each pair of filter cells is proportional to the partial pressure of the gas and is insensitive to changes in intensity of the IR source.
Dual-Beam Positive Filter
In the dual-beam positive-filter design, the IR energy from the source is split into two parallel beams: one passes through the sample gas, the other passes through a reference gas. A spinning blade passes through the beams and sequentially interrupts one, the other, then both. The two beams are optically focused on a single point, where a band pass optical filter selected at the absorption peak of the gas of interest is mounted over a single detector. As before, the ratios of the intensities of the sample and reference beams are proportional to the partial pressure of the gas.
Detectors of Infrared Radiation
To measure carbon dioxide, nitrous oxide, and sometimes anesthetic agents, a radiation-sensitive solid-state material, lead selenide, is commonly used as a detector. Lead selenide is quite sensitive to changes in temperature; it therefore is usually thermostatically regulated (cooled or heated) or temperature compensated.
Anesthetic agents, carbon dioxide, and nitrous oxide are sometimes measured with another detector called a Luft cell. This detector uses a chamber filled with gas that expands as IR radiation enters the chamber and is absorbed. A flexible wall of the chamber acts as a diaphragm that moves as the gas expands, and a microphone converts the motion to an electrical signal.
The signal processor converts the measured electrical currents to display gas partial pressure. First, the ratios of detector currents at various points in the spinning wheel’s progress, or from multiple detectors, are computed. Next, electronic scaling and filtering are applied. Finally, linearization according to a reference table for the point-by-point conversion from electrical voltage to gas partial pressure is accomplished by a microprocessor. Compensation for cross-sensitivity or interference between gases can be accomplished by the microprocessor after linearization.
Infrared Wavelength and Anesthetic Agent Specificity
IR analyzers must use a specific wavelength of radiation according to the absorbance peak of each gas to be measured. Early agent analyzers, such as the Datex Puritan-Bennett Anesthetic Agent Monitor ( Fig. 8-11 ), used a wavelength of 3.3 μm to measure the potent inhaled anesthetics. However, use of a single wavelength did not permit differentiation among these agents (see Fig. 8-7 ). When this system was used, the analyzer had to be programmed by the user for the particular agent being administered. This set the appropriate gain in the software program, and the displayed reading was then accurate for the one agent in use. Obviously, programming such an analyzer for the wrong agent, or the use of mixed agents, would lead to erroneous readings.

Modern IR analyzers are agent specific; that is, by measuring each agent with a unique set of wavelengths, they have the capability to both identify and quantify mixed agents in the presence of one another. Contemporary analyzers that can identify and quantify anesthetic agents incorporate individual wavelength filters in the range of 8 to 12 μm. An example is the Datex-Ohmeda Compact Airway Module (GE Healthcare) (see Figs. 8-2 and 8-9 ), which measures the absorption of the gas sample at seven different wavelengths selected using optical narrow band filters. In this analyzer module, the IR radiation detectors are thermopiles. Carbon dioxide and nitrous oxide are calculated from absorption measured at 3 to 5 μm ( Fig. 8-12 ). Identification and calculation of the concentrations of anesthetic agents are done by measuring absorption at five wavelengths in the 8- to 9-μm band and solving for the concentrations from a set of five equations, one for each agent ( Fig. 8-13 ). A schematic of a multiwavelength analyzer in which the beam of radiation is interrupted mechanically is shown in Figure 8-14 .



Sampling Systems and Infrared Analysis
Sidestream-sampling analyzers continuously withdraw between 50 and 250 mL/min from the breathing circuit through narrow-gauge sample tubing to the optical system, where the measurement is made. One of the disadvantages of sidestream monitors is the need to deal with liquid water and water vapor. Water vapor from the breathing circuit condenses on its way to the sample cuvette and can interfere with optical transmission. NAFION tubing, a semipermeable polymer that selectively allows water vapor to pass from its interior to the relatively dry exterior, is commonly used to eliminate water vapor. Also, a water trap often is interposed between the patient sampling catheter and the analyzer to protect the optical system from liquid water and body fluids (see Fig. 8-1 ). Filters integrated with the sampling tubing have replaced water traps in many of the currently available systems. Three different methods are available; these include 1) blocking the water, such as with a hydrophobic filter with large surface area (Oridion Medical, Jersusalem, IL), 2) absorbing and blocking, such as with a hydrophilic fibrous element followed by a hydrophobic plug (Philips Respironics, Wallingford, CT), and 3) active water removal with a hydrophilic wick, such as an elastomer, described as being able to “sweat” water collected from the gas sample flow to the outer surface of the cover (Nomoline, PhaseIn).
Infrared Photoacoustic Spectrometer
The photoacoustic spectrometer is similar to the basic IR spectrometer ( Fig. 8-15 ). IR energy is passed through optical filters that select narrow-wavelength bands that correspond to the absorption characteristics of the respired gases. Carbon dioxide is measured at a wavelength of 4.3 μm, nitrous oxide at 3.9 μm, and the potent inhaled agents at a wavelength between 10.3 and 13.0 μm. Evenly spaced windows are located along the circumference of a rotating wheel. The optical components are located astride the wheel along one of its radii. A series of IR beams pulse on and off at particular frequencies, according to the rate of rotation of the wheel and the spacing of the windows. The gas flowing through the measurement cuvette is exposed to the pulsed IR beams. As each gas absorbs the pulsating IR energy in its absorption band, it expands and contracts at that frequency, and resulting sound waves are detected with a microphone. The partial pressure of each gas in the sample is then proportional to the amplitude, or “volume,” of the measured sound.

The photoacoustic technique has the distinct advantage over other IR methods in that a simple microphone detector can be used to measure all the IR-absorbing gases. However, this device is sensitive to interference from loud noises and vibration. Also, because only one wavelength is used to measure the potent inhaled anesthetics, this monitor is unable to distinguish among the agents, which requires that it be programmed for the agent in use; also, erroneous readings might arise in the presence of mixed anesthetic agents. This technology was used in the Brüel and Kjær Anesthetic Gas Monitor 1304, and it is used currently in atmospheric trace gas monitors, such as the Innova 1412 (LumaSense Technologies, Santa Clara, CA).
Recent Technologies
Philips Respironics introduced a flexible monitoring interface, now known as CO 2 nnect & Go, which allows the user to choose, based on the patient and environment, which carbon dioxide–monitoring modality should be used, sidestream or mainstream. A complete measurement system is available for both mainstream (Capnostat 5) and sidestream (LoFlo, either external or internal format) modes of gas sampling.
Mainstream multigas IR analysis has recently been introduced by Phasein in their IRMA series of multigas analyzers. The IRMA mainstream probe measures IR light absorption at 10 different wavelengths to determine gas concentrations in the mixture ( Fig. 8-16 ). Adult, pediatric, and infant disposable airway adapters are available. In a bench study, the monitor was found to have a response time for carbon dioxide (96 vs. 348 ms) and oxygen (108 vs. 432 ms) that was significantly less than a contemporary sidestream-sampling gas monitor. The same miniaturized technology is used in Phasein’s EMMA Emergency Capnometer, a device that displays respiratory rate and incorporates apnea, high carbon dioxide, and low carbon dioxide audible and visual alarms ( Fig. 8-17 ).


Although Microstream (Oridion Medical) capnography is now used as a generic term to refer to a sampling flow rate of 50 mL/min, a rate now available from several vendors, it also encompasses the unique IR emission source. This approach is called molecular correlation spectroscopy (MCS), and it produces selective emission of a spectrum of discrete wavelengths, approximately 100 discrete lines in the 4.2- to 4.35-μm range, which match those for carbon dioxide absorption. This is compared with the broad IR emission spectrum of black body emitters used with conventional nondispersive IR technology and permits use of a smaller sample cell (15 μL). This technology has been adapted for use in portable carbon dioxide monitors.
Raman Spectroscopy
When light strikes gas molecules, most of the energy scattered is absorbed and reemitted in the same direction, and at the same wavelength, as the incoming beam (Rayleigh scattering). At room temperature, about one millionth of the energy is scattered at a longer wavelength, producing a so-called red-shifted spectrum. Raman scattering can be used to measure the constituents of a gas mixture. Unlike IR spectroscopy, Raman scattering is not limited to gas species that are polar. Carbon dioxide, oxygen, nitrogen, water vapor, nitrous oxide, and the potent volatile anesthetic agents all exhibit Raman activity. Monatomic gases such as helium, xenon, and argon, which lack intramolecular bonds, do not exhibit Raman activity.
The medical Raman spectrometer used a helium-neon laser (wavelength 633 nm, or 0.633 μm) to produce the incoming monochromatic light beam. The Raman scattered light is of low intensity and is measured perpendicular to the laser beam. The measurement cuvette is located in the cavity of the laser, so that the gas molecules are struck repeatedly by the beam ( Fig. 8-18 ). This results in enough Raman scattering to be collected and processed by the optical detection system. Photomultiplier tubes count the scattered photons at the characteristic Raman-shifted wavelength for each gas. Thus the Raman spectrometer measures the partial pressures of the gases in its measurement cuvette, and measurements are converted electronically to the desired units of measure and are displayed on the screen.

Raman spectroscopy is the principle of operation of the Ohmeda Rascal II monitor ( Fig. 8-19 ). The Rascal II Raman spectrometer has the same capabilities as the mass spectrometer; in particular, it is able to measure nitrogen for detection of air embolism, and it received a very favorable evaluation. Unfortunately, despite its obvious versatility, this monitor is no longer in production, although a number remain in use.

Water Vapor and Accuracy of Capnometers
Water vapor can be an important factor in the accuracy of a carbon dioxide analyzer. Most sidestream analyzers report ambient temperature and pressure dry (ATPD) values for P co 2 by using a water trap and water vapor–permeable NAFION tubing to remove water vapor from the sample. It has been recommended that carbon dioxide analyzers report their results at body temperature and pressure saturated (BTPS) so that end-tidal values are close to conventionally reported alveolar gas partial pressure. The error in reporting P co 2 at ATPD, when it should be reported at BTPS, is approximately 2.5 mm Hg. Carbon dioxide values reported in ATPD can be converted to BTPS by decreasing the dry gas reading by the fraction (P ATM − 47)/P ATM , where P ATM is the atmospheric pressure in mm Hg, and 47 mm Hg is the vapor pressure of water at 37° C.
Mainstream sampling analyzers naturally report readings at the breathing circuit conditions that are typically near BTPS. Depending on these conditions, a small decrease from body temperature may result in the analyzer reading slightly less than BTPS values. Condensation of water can affect the windows of the mainstream airway adapter and cause erroneous readings. These adapters are therefore heated or use coatings on the inside of the windows to prevent condensation.
Colorimetric Carbon Dioxide Detectors
Carbon dioxide in solution is acidic; pH-sensitive dyes can therefore be used to detect and measure its presence. A colorimetric carbon dioxide detector is designed to be interposed between the tracheal tube and the breathing circuit. Respired gas passes through a hydrophobic filter and a piece of filter paper visible through a plastic window. The originally described detector, the Fenem CO 2 Indicator (Engineered Medical Systems, Indianapolis, IN), consisted of a piece of filter paper permeated with an aqueous solution of metacresol purple, a pH-sensitive dye. Carbon dioxide from the exhaled breath dissolves in the solution, changing the color of the dye from purple to yellow; the degree of color change depends on the carbon dioxide concentration. On inspiration of carbon dioxide–free gas, carbon dioxide leaves the solution and the color of the indicator returns to purple.
A number of colorimetric devices are now commercially available for use in adult and pediatric patients. They may use other carbon dioxide–sensitive dyes and are calibrated to provide an approximate indication of expired carbon dioxide concentration that can be discerned by comparison of the indicator color with a graduated color scale printed on the device’s housing. For example, with the Easy Cap II (Covidien, Mansfield, MA), the color changes from purple to yellow to indicate 2% to 5% carbon dioxide (15 to 38 mm Hg) with each exhaled breath. On inspiration the color should change back to purple, indicating absence of carbon dioxide in the inspired gas ( Fig. 8-20 ). A permanent change in color may mislead the uneducated user. The following caution appears in the directions for use from the manufacturer: “Interpreting results before confirming six breath cycles can yield false results. Gastric distension with air prior to attempted intubation may introduce carbon dioxide levels as high as 4.5% into the Easy Cap detector if the endotracheal tube is misplaced in the esophagus. Initial Easy Cap detector color (yellow) may be interpreted as a false positive if read before delivery of six breaths.” The warnings also further include a statement that “reflux of gastric contents, mucus, edema fluid, or intratracheal epinephrine into the Easy Cap can yield persistent patchy yellow or white discoloration which does not vary with the respiratory cycle . Contamination of this type may also increase airway resistance and affect ventilation. Discard device if this occurs.”

This type of detector is intended to be used to confirm clinical signs of tracheal intubation when conventional capnography is not available. Both adult and pediatric versions are available. The newest Fenem carbon dioxide indicator is designed to be attached to the exhalation port (19 or 30 mm) of a self-inflating resuscitator bag, where it does not add dead space or resistance to flow ( Fig. 8-21 ).

Another version of the colorimetric carbon dioxide detector, the CO 2 nfirm Now carbon dioxide detector (Covidien), is marketed as a device to confirm intragastric placement of an orogastric or nasogastric tube to avoid intratracheal placement ( Fig. 8-22 ).

Oxygen Analyzers
In all contemporary anesthesia delivery systems, the fraction of inspired oxygen (Fi o 2 ) in an anesthesia breathing circuit is monitored by an oxygen analyzer. Two types of oxygen analyzers are in common use for monitoring: those based on a fuel or galvanic cell principle and the paramagnetic (Pauling) sensor. In the past, in addition to these methods, multigas analyzers that used mass spectroscopy or Raman spectroscopy to measure oxygen were also used.
Fuel Cell Oxygen Analyzer
The fuel cell ( Fig. 8-23 ), or galvanic cell, is basically an oxygen battery that consists of a diffusion barrier; a noble metal cathode, either gold mesh or platinum; and a lead or zinc anode in a basic, usually potassium hydroxide, electrolyte bath ( Fig. 8-24 ). The sensor is covered by an oxygen-permeable membrane and is exposed to the gas in the breathing circuit. Oxygen diffusing into the sensor is reduced to hydroxyl ions at the cathode in the following reaction:
O 2 + 2 H 2 O + 4 e − → 4 ( OH ) −
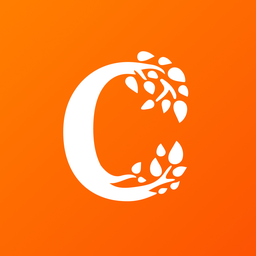
Full access? Get Clinical Tree
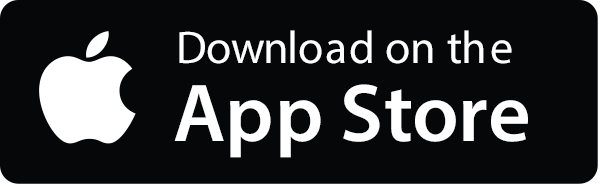
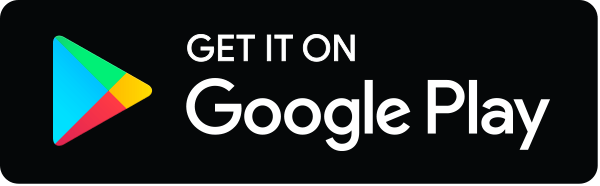