Respiratory Failure Part V: Extrapulmonary Causes of Respiratory Failure
Helen M. Hollingsworth
Melvin R. Pratter
Richard S. Irwin
The conditions that cause respiratory failure primarily by their effect on structures other than the lungs are discussed in this chapter. Severe impairment of the extrapulmonary compartment produces respiratory failure through the mechanism of hypoventilation (see Chapter 46), so the resultant respiratory failure is always hypercapnic. Extrapulmonary causes account for up to 17% of all cases of hypercapnic respiratory failure [1]. This chapter is organized to follow sequential sections of pathophysiology, diagnosis, differential diagnosis, and treatment.
Pathophysiology
The extrapulmonary compartment includes the (a) central nervous system (CNS), (b) peripheral nervous system, (c) respiratory muscles, (d) chest wall, (e) pleura, and (f) upper airway [2]. Because many conditions can cause extrapulmonary respiratory failure, it is helpful to categorize them according to the specific component affected by the disease process (Fig. 50.1). We have limited the discussion that follows to descriptions of the individual diseases and conditions that are most important to the topic of respiratory failure. They are summarized in Tables 50.1 through 50.4.
The pathophysiology of extrapulmonary respiratory failure is described in Chapter 45. Functionally, extrapulmonary disorders can lead to hypercapnic respiratory failure due to a decrease in normal force generation (e.g., CNS dysfunction, peripheral nervous system abnormalities, or respiratory muscle dysfunction) or an increase in impedance to bulk flow ventilation (e.g., chest wall and pleural disorders or upper airway obstruction) [3].
Diagnosis
General Considerations
Arterial hypercapnia in the presence of a normal alveolar–arterial oxygen tension [P(A–a)O2] gradient on room air is the sine qua non of extrapulmonary respiratory failure [4]. The normal gradient reflects the fact that in pure extrapulmonary failure distal gas exchange is normal, and the decrease in the partial pressure of arterial oxygen (PaO2) directly reflects the decrease in the partial pressure of alveolar oxygen (PAO2). A P(A–a)O2 gradient of less than 20 mm Hg in the presence of an elevated partial pressure of carbon dioxide (PaCO2) is, with few exceptions, diagnostic of extrapulmonary respiratory failure [5,6,7,8,9,10,11]. The main exception occurs in patients with chronic obstructive pulmonary disease (COPD) who have increasing hypercapnia [12]. Their P(A–a)O2 gradient can occasionally narrow to normal, probably related to substantial changes in the position of the alveolar and arterial points on the oxyhemoglobin dissociation curve related to ventilation–perfusion inequalities [12]. Thus, arterial hypercapnia with a normal P(A–a)O2 gradient is consistent with pure extrapulmonary respiratory failure, but a normal P(A–a)O2 cannot, by itself, rule out severe COPD.
Pulmonary parenchymal disease can also exist concomitantly with extrapulmonary dysfunction. For example, a patient with polymyositis can have respiratory muscle weakness in addition to interstitial pulmonary fibrosis. This may be suggested by the combination of hypercapnia and only mild-to-moderate widening of the P(A–a)O2 gradient. A gradient between 20 and 30 mm Hg in the presence of arterial hypercapnia should raise the suspicion that a significant element of extrapulmonary dysfunction may be present. It is also important to realize that even when the P(A–a)O2 gradient exceeds 30 mm Hg, some degree of extrapulmonary dysfunction can also be present in association with significant pulmonary impairment. For example, when hypercapnic respiratory failure results from an acute exacerbation of COPD, respiratory muscle fatigue often contributes to the development of carbon dioxide retention [13]. A less common example is the presence of a large abdominal ventral hernia in a patient with COPD. The resultant paradoxic breathing pattern can contribute significantly to abnormal gas exchange and increased dyspnea [14].
Decrease in Normal Force Generation
Because the inspiratory muscles generate the force that results in ventilation, any condition that directly or indirectly impairs respiratory muscle function can result in decreased force generation [3]. Dysfunction of the respiratory center, peripheral nervous system pathways, or the respiratory muscles themselves decreases the force available to produce ventilation. If this impairment is severe enough, the level of minute ventilation will be insufficient to clear the amount of carbon dioxide produced by ongoing metabolic processes, and hypercapnic respiratory failure results.
An acute decrease in CNS output sufficient to result in hypercapnic respiratory failure (e.g., acute narcotic overdose) is usually accompanied by obvious evidence of generalized CNS depression. In contrast, a chronic (e.g., primary alveolar hypoventilation) or episodic (e.g., central sleep apnea) cause of decreased impulse formation may present a much more difficult diagnostic dilemma. Tests to evaluate respiratory center drive, such as voluntary hyperventilation, carbon dioxide stimulation, or polysomnography, may be necessary to define the problem.
Peripheral nervous system dysfunction or primary weakness of the respiratory muscles is often indicated by the presence of certain suggestive clinical findings that vary depending on the
specific entity present (see following discussion). Respiratory muscle fatigue or weakness may be suspected clinically and documented using a number of tests designed to evaluate respiratory muscle function.
specific entity present (see following discussion). Respiratory muscle fatigue or weakness may be suspected clinically and documented using a number of tests designed to evaluate respiratory muscle function.
Symptoms are usually nonspecific; patients may report dyspnea on exertion, either when supine (bilateral diaphragmatic paralysis) or when upright (C5–6 quadriplegia). Reports of weakness in other muscle groups, difficulty swallowing, and change in voice volume or tone may be other clues. Physical findings of changes in the rate, depth, and pattern of breathing suggest stressed, fatigued, or weakened respiratory muscles. For example, an increased respiratory rate, a decreased tidal volume, and paradoxic inward motion of the anterior abdominal wall during inspiration may be observed. The latter finding indicates a failure of the diaphragm to contract sufficiently to descend and move the abdominal contents downward and the abdominal wall outward during inspiration. A breathing pattern that cycles between predominantly chest wall or predominantly abdominal wall motion, called respiratory alternans, represents the alternating contraction of intercostal and accessory muscles, on the one hand, and the diaphragm, on the other. The assumption is that these two muscle groups alternate in their contribution to the work of breathing, allowing one another to rest during periods of muscle overload or fatigue.
Two readily available tests can be useful diagnostically to help assess respiratory muscle function. First, measurement of maximal inspiratory and expiratory pressures at the mouth is easy to perform, noninvasive, and can accurately predict the development of hypercapnic respiratory failure due to decreased respiratory muscle force generation [15,16]. Arterial hypercapnia due to respiratory muscle weakness is generally not seen until the maximal inspiratory pressure is reduced to 30% or less of normal [15,16]. Although normal predicted values vary (primarily on the basis of age and gender [17,18]), a maximal inspiratory pressure less negative than -30 cm H2O is likely to be associated with arterial hypercapnia [16,19]. Maximal expiratory pressures are also reduced when there is respiratory muscle weakness, and in some neuromuscular disorders, the decrease may be even greater than that of the corresponding inspiratory pressure [16]. A maximal expiratory pressure of less than 40 cm H2O is generally associated with a poor cough and difficulty clearing secretions [19].
A second measurement that is valuable in predicting the development of arterial hypercapnia due to neuromuscular weakness is the vital capacity. It can be performed either in the pulmonary function laboratory or at the bedside. [15,19]. Although a vital capacity of less than 1 L, or less than 15 mL per kg of body weight is commonly associated with arterial hypercapnia [1,19], the vital capacity is a less sensitive predictor of arterial hypercapnia than is the maximal inspiratory pressure, particularly in patients with chest wall disorders such as kyphoscoliosis [16]. Significant arterial hypercapnia is unlikely to occur with an inspiratory pressure more negative than -30 cm H2O; however, arterial hypercapnia may be present with a vital capacity as high as 55% or as low as 20% of the predicted value [15,16].
The measurement of transdiaphragmatic pressures (Pdi) and diaphragmatic electromyograms (EMGs), although not commonly used clinically, may be helpful. An inspiratory effort associated with a Pdi consistently more than 40% of maximum predictably results in diaphragmatic fatigue [20]. Therefore, it follows that patients with diaphragmatic weakness and a reduced maximum Pdi are at risk for developing diaphragmatic fatigue and respiratory failure, even in the face of normal inspiratory pressure [20]. Similarly, a decrease of more than 20% from baseline in the high- to low-frequency ratio as measured by the diaphragmatic EMG indicates diaphragmatic fatigue and portends the development of hypercapnic failure [21,22].
Central Nervous System Dysfunction
The respiratory center, located in the brainstem, is composed of two main parts, the medullary center and the pneumotaxic center [23,24]: The medullary center is responsible for initiation and maintenance of spontaneous respiration, and the pneumotaxic center in the pons helps coordinate cyclic respiration. A decrease in central drive can occur due to a direct central loss of sensitivity to changes in PaCO2 and pH or a peripheral chemoreceptor loss of sensitivity to hypoxia as a result of CNS depressants, metabolic abnormalities, structural lesions, primary alveolar hypoventilation, and central sleep apnea (Table 50.1) [25,26,27,28,29,30,31,32,33,34,35,36,37,38,39,40,41,42,43].
Peripheral Nervous System Dysfunction
Disruption in impulse transmission from the respiratory center to the respiratory muscles can eventuate in respiratory failure. This disruption can be caused by spinal cord disease [44], anterior horn cell disease [45,46], peripheral neuropathy, or neuromuscular junction blockade [19] (Table 50.2) [5,25,44,45,46,47,48,49,50,51,52,53,54,55,56,57,58,59,60,61,62,63,64,65,66,67,68,69,70,71,72,73,74,75,76,77,78,79,80,81,82,83,84,85,86,87,88]. Denervation of the inspiratory muscles may occur as part of a generalized process (e.g., Guillain–Barré syndrome, myasthenia gravis [19]) or as an isolated abnormality (e.g., phrenic nerve palsy secondary to hypothermic cardioplegia during cardiac surgery [67,89]).
Peripheral nervous system dysfunction severe enough to produce hypercapnic respiratory failure is always associated with pulmonary function test findings of a reduced vital capacity (usually less than 50% of the predicted value [15,19]) and markedly decreased maximal inspiratory and expiratory pressures (usually 30% of the predicted pressures [15,19,55]). This type of respiratory failure is characterized by an ineffective cough and a high incidence of aspiration, atelectasis, and pneumonia [5].
The effect on the respiratory system of interruption of CNS impulse transmission due to spinal cord abnormalities is highly dependent on the level of the injury [44,47]. A lesion at the C3
vertebral level or above abolishes both diaphragmatic and intercostal activity, leaving only some residual accessory muscle function [47]. The result is severe hypercapnic respiratory failure. Acute spinal cord lesions at the C5 and C6 levels produce an immediate fall in the vital capacity to 30% of the predicted value, due to loss of intercostal and abdominal muscle function [44]. This is associated with a limitation of both inspiratory capacity and active expiration. Within approximately 3 months of injury, however, the denervated muscles become stiff, which enables improved diaphragmatic efficiency. This improvement usually leads to an increase in the vital capacity to 50% to 60% of normal. Midthoracic spinal cord lesions have relatively little impact on respiratory muscle function because they principally affect the abdominal muscles, resulting in only a limitation of active expiration and cough [5,47].
vertebral level or above abolishes both diaphragmatic and intercostal activity, leaving only some residual accessory muscle function [47]. The result is severe hypercapnic respiratory failure. Acute spinal cord lesions at the C5 and C6 levels produce an immediate fall in the vital capacity to 30% of the predicted value, due to loss of intercostal and abdominal muscle function [44]. This is associated with a limitation of both inspiratory capacity and active expiration. Within approximately 3 months of injury, however, the denervated muscles become stiff, which enables improved diaphragmatic efficiency. This improvement usually leads to an increase in the vital capacity to 50% to 60% of normal. Midthoracic spinal cord lesions have relatively little impact on respiratory muscle function because they principally affect the abdominal muscles, resulting in only a limitation of active expiration and cough [5,47].
Table 50.1 Respiratory Failure Caused By Central Nervous System Dysfunction | ||||||||||||||||||||||||||||||||||||||||||||||||||||||||||||
---|---|---|---|---|---|---|---|---|---|---|---|---|---|---|---|---|---|---|---|---|---|---|---|---|---|---|---|---|---|---|---|---|---|---|---|---|---|---|---|---|---|---|---|---|---|---|---|---|---|---|---|---|---|---|---|---|---|---|---|---|
|
Most spinal cord diseases interrupt impulse transmission, resulting in respiratory muscle weakness, but two notable exceptions exist: tetanus and strychnine poisoning. In both conditions, inhibitory influences at the spinal cord and anterior horn cell level decrease [51,52], causing a simultaneous increase in motor activity to groups of muscles that are normally antagonistic to one another. This results in intense muscle spasms, including involvement of the upper airway muscles, diaphragm, and intercostal muscles. The repetitive spasms and episodes of apnea, result in severe arterial hypoxemia, hypercapnia, and metabolic acidosis [51,52].
Diseases that involve the anterior horn cells of the spinal cord interrupt efferent impulse transmission. Amyotrophic lateral sclerosis (ALS) is the most common anterior horn cell disease causing respiratory failure [5,45,47]. In most cases of ALS, the patient develops segmental muscular atrophy, weakness of the distal extremities, hyperreflexia, fasciculations, and bulbar paralysis [45]. Although respiratory failure usually develops late in the course of the disease, it may rarely be the presenting manifestation [45]. Repetitive episodes of aspiration secondary to bulbar dysfunction may contribute to respiratory impairment [5]. It has been speculated that antecedent poliomyelitis may be involved in some cases of amyotrophic lateral sclerosis [53]. A postpolio syndrome, characterized by new, slowly progressive muscle weakness, may develop years after recovery from acute poliomyelitis [57].
Polyneuropathies with prominent motor neuron involvement, (e.g. Guillain–Barré syndrome) can affect the respiratory nerves and lead to respiratory failure (see Chapter 175) [25]. Symmetric, predominantly distal muscle weakness with absent tendon reflexes is the typical presentation [25]. In one series of patients with Guillain–Barré syndrome, 28% required mechanical ventilatory assistance. The average duration of mechanical ventilation was 9 weeks (range, 3 weeks to 7 months). Although the mortality rate is generally low, 21% of hospitalized patients died in one series [90]. Guillain–Barré syndrome may be associated with autonomic dysfunction including new-onset hypertension (57%), sinus tachycardia (50%), postural hypotension (43%), or facial flushing (25%) [90].
Dinoflagellate toxin poisoning, from red tide-contaminated shellfish and ciguatera-contaminated reef and other fish, is a dramatic but uncommon cause of peripheral neuropathy resulting that can produce respiratory failure [61,62,63,64,65,66]. The responsible agents are heat-stable neurotoxins that interfere with action potential propagation along peripheral nerves. During the warm summer months, the dinoflagellates that produce the toxins proliferate and are ingested by shellfish and fish. The
clinical picture is virtually pathognomonic. Within 30 minutes of ingesting contaminated shellfish, tingling and numbness of the face, lips, and tongue develop. Paresthesias and muscle weakness follow, with rapid progression to limb and respiratory muscle paralysis [62,63]. Multiple-case presentations from one source of exposure are common.
clinical picture is virtually pathognomonic. Within 30 minutes of ingesting contaminated shellfish, tingling and numbness of the face, lips, and tongue develop. Paresthesias and muscle weakness follow, with rapid progression to limb and respiratory muscle paralysis [62,63]. Multiple-case presentations from one source of exposure are common.
Table 50.2 Respiratory Failure Caused By Peripheral Nervous System Dysfunction | ||||||||||||||||||||||||||||||||||||||||||||||||||||||||||||||||||||||||||||||||||||||||||||||||||||||||||||
---|---|---|---|---|---|---|---|---|---|---|---|---|---|---|---|---|---|---|---|---|---|---|---|---|---|---|---|---|---|---|---|---|---|---|---|---|---|---|---|---|---|---|---|---|---|---|---|---|---|---|---|---|---|---|---|---|---|---|---|---|---|---|---|---|---|---|---|---|---|---|---|---|---|---|---|---|---|---|---|---|---|---|---|---|---|---|---|---|---|---|---|---|---|---|---|---|---|---|---|---|---|---|---|---|---|---|---|---|
|
Peripheral phrenic nerve palsies can contribute to or cause hypercapnic respiratory failure, particularly if they are bilateral [91]. Bilateral phrenic nerve palsies have been described as an uncommon complication of hypothermia used for cardioplegia during cardiac surgery (particularly when ice slush is used) [67], trauma [67,91], a variety of neurologic diseases (e.g., poliomyelitis and Guillain–Barré syndrome) [67,68,91], Charcot–Marie–Tooth disease [70], intrathoracic malignancies [92], and as a part of a paraneoplastic syndrome [93]. Bilateral diaphragmatic paralysis can also be idiopathic [94]. The characteristic clinical findings of bilateral diaphragmatic paralysis are severe orthopnea and marked abdominal paradoxic in the supine position [69,89,91,95]. Fluoroscopy during a sniff test is more helpful in identifying unilateral than bilateral diaphragm paralysis, as upward motion of the ribs during inspiration can make the diaphragm appear to descend. The diagnosis of diaphragmatic paralysis is usually confirmed by transdiaphragmatic pressure measurements that reveal a minimal or absent Pdi gradient [91]. Electromyography of the diaphragm and phrenic nerve conduction velocity studies may also be helpful.
Several other causes of peripheral neuropathy can involve the efferent pathways to the respiratory muscles including diphtheria, herpes zoster infection, tick paralysis, acute
intermittent porphyria, beriberi, and a variety of metabolic disorders [25]. Respiratory failure associated with diphtheria is of delayed onset, usually occurring 4 to 6 weeks after the onset of illness [25]. Tick paralysis is seen mainly in children in whom the presence of the tick goes unnoticed for 5 to 6 days [25]. In acute intermittent porphyria, respiratory involvement may be a slowly progressive process or may cause an abrupt deterioration in respiratory function due to bilateral phrenic nerve paralysis [37]. Myasthenia gravis [19], botulism [84,85,86], organophosphate poisoning [25], and a variety of drugs can produce neuromuscular blockade that results in respiratory failure [76]. Although patients with myasthenia gravis typically show signs of obvious muscle weakness and rapid fatigability, particularly of the cranial muscles, before the development of respiratory failure, acute respiratory failure is occasionally a presenting manifestation [25,72]. More commonly, respiratory failure complicates myasthenia gravis after surgical procedures, following the institution of glucocorticoid therapy, or, as a result of under- or overtreatment with anticholinesterase medications [19].
intermittent porphyria, beriberi, and a variety of metabolic disorders [25]. Respiratory failure associated with diphtheria is of delayed onset, usually occurring 4 to 6 weeks after the onset of illness [25]. Tick paralysis is seen mainly in children in whom the presence of the tick goes unnoticed for 5 to 6 days [25]. In acute intermittent porphyria, respiratory involvement may be a slowly progressive process or may cause an abrupt deterioration in respiratory function due to bilateral phrenic nerve paralysis [37]. Myasthenia gravis [19], botulism [84,85,86], organophosphate poisoning [25], and a variety of drugs can produce neuromuscular blockade that results in respiratory failure [76]. Although patients with myasthenia gravis typically show signs of obvious muscle weakness and rapid fatigability, particularly of the cranial muscles, before the development of respiratory failure, acute respiratory failure is occasionally a presenting manifestation [25,72]. More commonly, respiratory failure complicates myasthenia gravis after surgical procedures, following the institution of glucocorticoid therapy, or, as a result of under- or overtreatment with anticholinesterase medications [19].
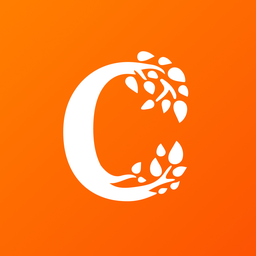
Full access? Get Clinical Tree
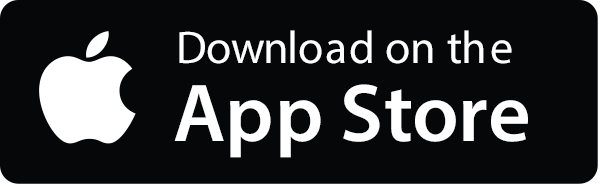
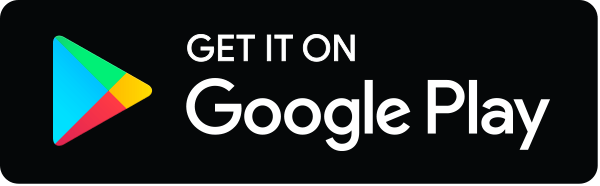