Renal Replacement Therapy in the Intensive Care Unit
Glenn Kershaw
Matthew J. Trainor
Pang-Yen Fan
Introduction
Rapid deterioration of kidney function in acutely ill patients is common and potentially catastrophic. Acute kidney injury (AKI) occurs in up to 70% of patients admitted to the intensive care unit (ICU) and is associated with a twofold increase in the already high mortality rate for this population. Medical therapy is often inadequate for management of the metabolic disturbances and fluid overload that complicate AKI. In this setting, renal replacement therapy (RRT) is essential to the survival of the patient. In addition, patients with end-stage renal disease (ESRD) have high rates of hospitalization, particularly for cardiovascular disease and infection. These patients often require ICU care including RRT.
We are now entering the fifth decade of providing dialysis support to ICU patients with AKI. Despite many technical advances, mortality remains alarmingly high (40% to 60%). The high death rate is largely due to the severity and the array of nonrenal organ system dysfunction, as mortality for patients with AKI is now primarily due to multiorgan system failure (MOSF). Accumulating evidence suggests that the acutely injured kidney may in turn injure distant organs and that some forms of RRT may prevent MOSF [1].
The objectives of modern day RRT now extend beyond correction of metabolic disturbances and volume overload to include facilitation of nutritional support and drug therapy, optimization of volume status, and even promotion of nonrenal organ system recovery. Treatment strategies have shifted from reactive to proactive approaches, leading to a trend toward earlier initiation of dialysis. For example, with growing recognition that fluid overload increases mortality and that volume control can improve outcomes [2,3,4], RRT is applied more aggressively to prevent volume overload. An important principle of renal support strategies is that of “capacity (supply) and demand mismatch.” Critically ill patients have increased “demand” for renal function. Such patients often generate increased solute from their hypercatabolic metabolism and intensive nutritional support. In addition, they receive enormous amounts of fluid because of medications, blood products, enteral and parenteral nutrition, and volume resuscitation. The stress of high solute and fluid loads may overwhelm the capacity of even a minimally injured kidney. Furthermore, the limited “supply” of renal function from kidneys already compromised by AKI is further reduced by tenuous hemodynamics and endogenous/exogenous renal pressor activity. RRT expands the limited capacity of the injured kidney to match the high fluid and solute demand, thereby restoring balance [5].
Principles of Solute Clearance and Fluid Removal by Dialytic Techniques
Dialysis therapies involve the movement of solute and plasma water across a semipermeable membrane separating a blood compartment and a dialysate compartment. For intermittent hemodialysis (IHD) and continuous renal replacement therapies (CRRT), this process occurs within a cartridge called a hemofilter or hemodialyzer as shown in Figure 75.1. For peritoneal dialysis (PD), the peritoneum serves as the semipermeable membrane separating blood in the mesenteric vasculature from the dialysate in the peritoneal cavity. Characteristics such as membrane thickness and pore dimensions determine the size and transfer rate of molecules that move between the blood and dialysate.
Removal of solute and water in RRT may occur by diffusion or convection. Diffusion involves movement of solute down a concentration gradient, that is, from areas of high concentration to low concentration. Conversely, water will move from an area of low osmolality to an area of high osmolality. Solute molecules have kinetic energy and move in solution. They collide with one another and with water molecules resulting in an even dispersion throughout the solution. Dialysis is diffusion across a semipermeable membrane. When solute in
motion encounters a membrane pore of sufficient dimensions, it moves through the membrane into the adjacent compartment. Small molecules with greater molecular velocity are more readily cleared than are larger molecules even if both fit through the membrane pore. A high concentration of solute in a given compartment favors a high frequency of membrane collisions and passage through the pore. Water will also pass easily through the membrane to the compartment with higher osmolality. Large shifts of water may pull some solute through the membrane, a phenomenon known as solvent drag. In a static system, net transfer (dialysis) ceases when solute concentrations equilibrate in the compartments. For RRT, blood and dialysate are continuously replenished to maintain the high concentration gradients favoring maximum transfer of solute and water.
motion encounters a membrane pore of sufficient dimensions, it moves through the membrane into the adjacent compartment. Small molecules with greater molecular velocity are more readily cleared than are larger molecules even if both fit through the membrane pore. A high concentration of solute in a given compartment favors a high frequency of membrane collisions and passage through the pore. Water will also pass easily through the membrane to the compartment with higher osmolality. Large shifts of water may pull some solute through the membrane, a phenomenon known as solvent drag. In a static system, net transfer (dialysis) ceases when solute concentrations equilibrate in the compartments. For RRT, blood and dialysate are continuously replenished to maintain the high concentration gradients favoring maximum transfer of solute and water.
Convection involves the transfer of solute across a semipermeable membrane driven by a hydrostatic pressure gradient. Those solutes small enough to pass through pores are swept along with water by solvent drag. The membrane acts as a sieve, retaining molecules that exceed the pore size. All filtered solutes below the membrane pore size are removed at rates proportionate to their concentration. The convective removal of fluid in this manner is termed hemofiltration or sometimes ultrafiltration. This technique does not change the plasma concentration of small solutes (blood urea nitrogen [BUN], creatinine, electrolytes, glucose), since water is removed in proportion to solute. In contrast, the concentration of larger molecules (albumin) and formed elements (hematocrit) increase as they are sieved off by the smaller membrane pores. Thus, the chemical composition of the filtrate (often referred to as ultrafiltrate) is almost identical to that of the plasma except for the absence of large molecules such as albumin. Some thought leaders opt for convection-based RRT because of greater clearance of medium-sized and large molecules than with diffusion-based techniques.
Overview of Dialysis Modalities
The general features of different dialysis modalities are summarized in Table 75.1.
Table 75.1 Dialysis Modalities | ||||||||||||||||||||||||||||||||||||||||||||||||
---|---|---|---|---|---|---|---|---|---|---|---|---|---|---|---|---|---|---|---|---|---|---|---|---|---|---|---|---|---|---|---|---|---|---|---|---|---|---|---|---|---|---|---|---|---|---|---|---|
|
Intermittent Hemodialysis
Intermittent hemodialysis is the standard form of RRT for the majority of stable ESRD patients in the United States. In IHD, blood is circulated through a dialysis machine and hemodialysis cartridge and then returned to the patient. Utilizing diffusion (principally for solute clearance) and convection (principally for ultrafiltration), this highly efficient modality provides rapid solute and volume removal but requires both specialized equipment and trained staff. Both blood and dialysate are pumped through the hemofilter at high flow rates. The dialysate flow is countercurrent to blood flow to maximize concentration gradients throughout the course of the filter (Fig. 75.1). Diffusion of solute across the filter is bidirectional. Urea, creatinine, and potassium move from plasma to dialysate, whereas bicarbonate and usually calcium diffuse in an opposite path (Fig. 75.2).
Standard dialysis machines can also perform isolated ultrafiltration which results in fluid removal but does not significantly alter the chemical composition of plasma. During ultrafiltration, the dialysis machine pumps only blood, but not dialysate through the hemofilter. This process generates a hydrostatic pressure gradient across the hemofilter membrane, resulting in convective fluid removal. However, no dialysate is used, so there is no diffusive solute clearance. Isolated ultrafiltration is typically used when volume overload is the sole concern.
Solute clearance can be adjusted changing the dialyzer size and membrane, blood and dialysate flow, and dialysis time as detailed later in this chapter. Fluid removal can be adjusted by changing the hydrostatic pressure gradient between the blood and dialysate compartments within the hemofilter, an automated process performed by the dialysis machine. Although this technique is commonly used in the ICU setting, the rapid shifts in solute and fluid can precipitate hemodynamic instability and may therefore be less suitable for critically ill patients. IHD treatments are typically performed for several hours three to four times per week. However, since the technique is labor and resource intensive, more frequent treatments may be limited by staffing and cost.
Peritoneal Dialysis
Peritoneal dialysis is the main form of RRT for approximately 5% to 10% of ESRD patients in the United States. In PD, dialysate is instilled into the peritoneal cavity. Through diffusion, solute and volume enter the dialysate, which is periodically drained and replaced with fresh dialysate. Solute clearance is adjusted by altering the volume of dialysate or varying the duration of each “dwell” (the interval between dialysate exchanges). More frequent exchanges will enhance solute removal, provided there is sufficient time between dialysate instillation and drainage to permit diffusion across the peritoneum. Volume is removed by maintaining a high dialysate osmolality through a high concentration of dextrose. This osmolar gradient results in the movement of water into the peritoneal cavity and also contributes to solute clearance through solvent drag. Fluid removal is adjusted by altering the dialysate dextrose content.
PD can be performed as a series of manual dialysate exchanges done during the day (chronic ambulatory peritoneal dialysis or CAPD) or through automated exchanges utilizing a PD machine (cycler) typically done at night (continuous cycled peritoneal dialysis or CCPD). For CAPD, dialysate is changed every 4 to 6 hours with a longer overnight “dwell.” For CCPD, the exchanges are typically done every 2 to 3 hours through the night, and the abdomen is often left empty or with only a small volume of dialysate (sometimes called a “cushion”) during the day. PD is much less efficient than IHD but is better tolerated hemodynamically, since solute and fluid shifts occur gradually. In the ICU setting, PD is generally reserved for ESRD patients who are already maintained on this modality. PD is generally not used for AKI because of technical difficulty in establishing dialysis access (discussed later in this chapter) as well as the
low efficiency of solute clearance. Furthermore, instillation of 1 to 2 L of dialysate into the peritoneal cavity can impair ventilation in patients with compromised oxygenation, particularly in the setting of abdominal distention or ileus.
low efficiency of solute clearance. Furthermore, instillation of 1 to 2 L of dialysate into the peritoneal cavity can impair ventilation in patients with compromised oxygenation, particularly in the setting of abdominal distention or ileus.
Continuous Renal Replacement Therapies
The continuous renal replacement therapies encompass a family of dialytic modalities that vary in their mode of solute removal and duration of treatment, but share the characteristic of slow solute and volume removal maintained over an extended period of time rather than high clearances over 3 to 4 hours as with IHD. Although less efficient than IHD, CRRT provides much higher clearances than PD. Like PD, these techniques are better tolerated hemodynamically than IHD, since solute and volume are removed gradually. These modalities require intensive monitoring and necessitate ICU admission. They are widely used in the critical care setting. CRRT can be performed by a number of methods detailed later in the chapter. The operating parameters of different CRRT systems are summarized in Table 75.2. A schematic of the circuitry of the different techniques is presented in Figure 75.3.
Continuous Arteriovenous Hemofiltration, Hemodialysis, and Hemodiafiltration
Arteriovenous (AV) systems were used at the dawn of CRRT. AV systems relied on the pressure gradient between the arterial and venous circulation to drive ultrafiltration across the hemofilter membrane. However, variations in arterial pressure led to inconsistent rates of ultrafiltration and solute clearance. AV systems also required the placement and long-term maintenance of large bore arterial catheters at the femoral site. With the development of reliable double-lumen venous catheters and advanced pump-driven venovenous systems, these modalities have largely fallen out of favor.
Continuous Venovenous Hemofiltration, Hemodialysis, and Hemodiafiltration
In CRRT, solute removal is achieved by convection, diffusion, or a combination of these methods. Continuous venovenous hemofiltration (CVVH) is a purely convective technique in which a pump system drives blood through the hemofilter and generates ultrafiltration rates of 1 to 4 L per hour. Blood flow rate is generally lower than with IHD and dialysate is not used. Solute clearance is achieved by replacing these large volumes of ultrafiltrate with fluid that does not contain the solutes targeted for removal (e.g., urea and potassium). Solute clearance and volume removal are adjusted by altering the ultrafiltration rate and the rate of infusion of replacement fluid (RF). The administration of RF maintains fluid balance and lowers the plasma concentration of solute by dilution. RF can be infused before or after the filter along the course of the dialyzer circuit (Fig. 75.3).
Table 75.2 Comparison of RRT Modalities | |||||||||||||||||||||||||||||||||||||||||||||||||||||||||||||||
---|---|---|---|---|---|---|---|---|---|---|---|---|---|---|---|---|---|---|---|---|---|---|---|---|---|---|---|---|---|---|---|---|---|---|---|---|---|---|---|---|---|---|---|---|---|---|---|---|---|---|---|---|---|---|---|---|---|---|---|---|---|---|---|
|
In diffusion-based techniques such as continuous venovenous hemodialysis (CVVHD), a dual pump system drives both blood and dialysate through the hemofilter. Dialysate flow and blood flow rates are typically much lower than with IHD. The technique creates less ultrafiltrate (2 to 5 L per day) than CVVH, since the infusion of dialysate lowers the pressure gradient across the hemofilter membrane. As with IHD, diffusion of solute across the filter is bidirectional (Fig. 75.2). No RF is administered. In CVVHD, the flow of dialysate through the filter is countercurrent to the flow of blood, but the dialysate flow rate is significantly slower (1 to 2 L per hour = 17 to 34 mL per minute) than the blood flow rate (100 to 200 mL per minute). This disparity permits full equilibration of plasma urea across the membrane and complete saturation of dialysate.
For all forms of CRRT, the effluent volume (Qef) relates directly to solute clearance and is a therapeutic target to assure dosing adequacy. The effluent volume is the product of the filtration process. It comprises the ultrafiltrate in CVVH, the “spent” (equilibrated) dialysate in CVVHD, and the combination of ultrafiltrate and spent dialysate in continuous venovenous hemodiafiltration (CVVHDF). Since urea is freely filtered in CVVH, its concentration in the ultrafiltrate is identical to that of plasma. Thus, 48 L of ultrafiltrate (effluent) represents 48 L of plasma fully cleared of urea. Similarly, in CVVHD, the effluent (spent dialysate) is fully saturated with urea. Each liter of spent dialysate reflects a liter of plasma fully cleared of urea. In diffusive systems, blood flow has little impact on clearance at low dialysate flow (1 to 2 L per hour) but increasing impact as dialysate flow increases.
CVVHDF combines diffusion and convection into a single procedure. Dialysate is infused at 1 to 2 L per hour to boost the convective clearance generated by high (1 to 2 L per hour) ultrafiltration rates. RF is needed to offset the high rate of ultrafiltration. Historically, CVVHDF was developed to overcome clearance limitations posed by the older generation of CRRT equipment which limited both dialyzer blood and dialysate flow. However, current CRRT equipment delivers blood flow at 400 mL per minute and dialysate flow at up to 10 L per hour. Large bore (13 French) catheters are increasingly used to support high flow systems. These advances allow high volume ultrafiltrate generation with less complex CVVH or CVVHD systems and have called the role of CVVHDF into question.
Slow Continuous Ultrafiltration
In slow continuous ultrafiltration (SCUF), a pump system maintains low blood flow (usually no more than 100 mL per minute) through a hemofilter and generates low rates of ultrafiltration (typically 100 to 300 mL per hour). This modality provides volume removal but does not alter the chemistry of plasma, since water is removed in proportion to solute. Compared with other CRRT modalities, SCUF is a low intensity nursing procedure. The procedure is often used in settings of severe volume overload with acceptable chemistries. At our institution, SCUF is often employed as an adjunct to IHD in the hemodynamically stable, volume overloaded patient.
Sustained Low Efficiency Dialysis
Hybrid therapies apply the CRRT principle of low solute clearance over an extended, but not continuous, period of time. Sustained low efficiency dialysis (SLED) is better tolerated hemodynamically than IHD and can be performed with either standard hemodialysis machines or with CRRT equipment. Lower blood flow (100 to 200 mL per minute) and dialysate flow (100 mL per minute) rates achieve adequate diffusive solute clearance and convective volume removal over a typical 8- to 12-hour session. SLED done with standard hemodialysis machines expands the clinical utility of these devices, but generally requires the presence of a trained dialysis nurse. However, this modality can be done with a CRRT machine by an ICU nurse. There are no data comparing SLED outcomes with either IHD or CRRT.
Technical Considerations for Renal Replacement Therapy
Anticoagulation
Hemofilter fibers are prone to thrombosis, as removal of fluid through ultrafiltration leads to hemoconcentration at the distal end of the dialyzer. As the filtration fraction (FF), that is, the proportion of plasma flow that is filtered, increases, the risk of filter thrombosis also rises. The FF can be calculated as follows:

Thus, higher rates of ultrafiltration, especially if coupled with low blood flows, predispose to hemofilter thrombosis. Poor filter performance and filter clotting increases sharply at FF greater than 20%. Higher blood flow rates permit greater rates of fluid removal, since hemoconcentration within the filter is limited by the short transit time of blood through the dialysis cartridge.
Hemodialysis can generally be performed without anticoagulation. The high blood flows used with this technique permit adequate solute clearance and ultrafiltration with limited risk of dialyzer thrombosis [6]. However, IHD without anticoagulation also necessitates frequent saline flushes through the hemodialyzer to help maintain fiber patency and therefore is more labor intensive than standard IHD. In addition, packed red blood cell transfusions cannot be infused through the arterial line of the dialyzer circuit, since the resulting increase in hematocrit will lead to hemofilter clotting.
However, with CCRT, blood flow rates are typically low and ultrafiltration rates high, especially for CVVH, and filter thrombosis is a significant barrier to effective implementation of these therapies. One approach called predilutional hemofiltration involves infusion of RF into the CRRT circuit at a point before the filter, thus lowering the hematocrit through dilution. As a result, a higher ultrafiltration rate may be achieved without compromising filter life. However, prefilter RF also dilutes the solute concentration of blood entering the filter and reduces effective clearance. With this approach, the target effluent volume should be increased by 25% to compensate for the dilutional effect. Other CRRT parameters such as blood and ultrafiltration rate must be adjusted to compensate for this inefficiency.
Anticoagulation, typically with unfractionated heparin, is generally necessary to maintain hemofilter patency with CRRT, especially for principally convective modalities such as CVVH. After an initial bolus of 1,000 to 2,000 units, a continuous infusion of approximately 10 units per kg per hour is adjusted to maintain the partial thromboplastin time in the venous line of the blood circuit at 1.5 to 2 times control. However, heparin infusions do result in some systemic anticoagulation and may be contraindicated in patients with active hemorrhage or heparin-induced thrombocytopenia (HIT).
Despite theoretical advantages, low-molecular-weight heparins do not appear to offer any significant advantages in efficacy or safety over unfractionated heparin for RRT [7]. In addition, these agents are more costly and their anticoagulant effects more difficult to monitor.
For patients with active hemorrhage, regional anticoagulation limited to the CRRT blood circuit is preferred. Citrate regional anticoagulation is widely used and has become the primary mode of anticoagulation in many centers. Citrate infused in the arterial limb of the CRRT circuit prevents hemofilter thrombosis by chelating calcium, a critical component of the clotting cascade. Calcium chloride infused into the venous line of the system restores normal systemic calcium levels. This approach appears to reduce the risk of hemorrhage and extend hemofilter patency [8]. In addition, citrate can be used for patient with HIT.
Serum and ionized calcium levels must be carefully monitored, especially in patients with significant liver dysfunction, and the calcium infusion appropriately adjusted. Citrate is hepatically metabolized into bicarbonate and can cause metabolic alkalosis. In the setting of hepatic failure, citrate accumulation results in elevated serum but low ionized calcium levels, reflecting increased circulating calcium bound to citrate. Trisodium citrate solution, typically used in this form of anticoagulation, may also cause hypernatremia.
Other methods of regional anticoagulation such as prostacyclin infusion or heparin reversal with protamine have been less successful. Prostacyclin, an arachidonic acid metabolite, has a half-life of only 3 to 5 minutes and inhibits platelet aggregation. However, it induces vasodilatation and is associated with hypotension and it is costly. Protamine binds and neutralizes heparin, but infusions are technically complex and may be associated with rebound bleeding.
Anticoagulation is unnecessary for PD. However, intraperitoneal fibrin can occlude the dialysis catheter. If fibrin clots are noted in the dialysate, heparin (1,000 units) should be added to each PD exchange for several days. Intraperitoneal heparin is not absorbed and will not cause systemic anticoagulation.
Blood Flow Rate
Maximal urea clearances with standard dialyzers require blood flows of approximately 400 mL per minute through the hemofilter. However, when dialysis is initiated for ESRD, blood flow rates begin at 200 to 250 mL per minute and are increased incrementally over several sessions. The low blood flow limits the efficiency of the dialysis and prevents rapid solute and water shifts that can precipitate complications including delirium, seizures, and dyspnea, collectively known as the dysequilibrium syndrome. With AKI, high blood flow rates (400 mL per minute) may be used immediately unless the BUN has been markedly elevated for a prolonged period (e.g., > 100 mg per dL for > 3 days). Blood flow rates for CRRT can vary from 100 to 400 mL per minute, but dysequilibrium syndrome is not a concern because solute and fluid removal are much less efficient than with IHD. For diffusive CRRT modalities such as CVVHD, dialysate flows are so low that solute concentrations fully equilibrate between the blood and dialysate compartments of the hemofilter. Therefore, increases in blood flow do not appreciably enhance solute clearance for these forms of RRT.
Dialysate Flow Rate
The dialysate flow rate is typically fixed or has very limited variability (500 to 800 mL per minute) in most hemodialysis machines. These rates are sufficiently high so that changes in dialysate flow have relatively little impact on IHD clearances. However, since dialysate flow rates are much lower with CRRT, increases in dialysate flow can significantly enhance solute removal. Thus, change in dialysate flow rate is an important adjustment to achieve adequate clearances with these modalities. With PD, clearances can also be increased by increasing total dialysate volumes, either by instilling more dialysate with each exchange or by increasing the frequency of exchanges. The maximal of volume per exchange is limited by abdominal discomfort and/or respiratory compromise, and high exchange volumes can predispose to leakage of dialysate from the catheter tunnel. Exchanges done more often than every 2 hours provide little additional solute clearance, as shorter dwell time limits diffusion across the peritoneum.
Dialyzer Membrane
Most hemodialyzers and hemofilters are constructed as cylinders containing hollow fibers composed of a semipermeable membrane (Fig. 75.1). The surface area of the membrane depends on the number and length of these fibers. Membrane surface area affects solute clearance and ultrafiltration. Membrane size or surface area varies with the specific model of hemodialyzer or hemofilter. Bigger dialysis cartridges are used for large patients or those needing high solute clearances. Children usually require specially downsized hemofilters.
The hemofilter membrane may consist of nonorganic, synthetic compounds (e.g., polysulfone, polyacryl nitrate) or cellulose-derived materials (cellulosic membranes). In vitro exposure of blood to cellulosic membranes leads to complement activation and leukocyte adherence and these membranes are categorized as bioincompatible. Synthetic membranes are inert and are termed biocompatible. A seminal randomized trial reported better survival and higher rates of recovery
from AKI among patients dialyzed on biocompatible membranes [9]. This outcome advantage was challenged in subsequent trials and meta-analyses [10]. Modern day cellulosic membranes claim biocompatibility, since the offending, complement-activating moiety (hydroxyl group) is now buried deep within the membrane. Regardless, we recommend exclusive use of synthetic (biocompatible) dialyzers in patients with AKI. The cost differential between synthetic and cellulosic membranes is minimal.
from AKI among patients dialyzed on biocompatible membranes [9]. This outcome advantage was challenged in subsequent trials and meta-analyses [10]. Modern day cellulosic membranes claim biocompatibility, since the offending, complement-activating moiety (hydroxyl group) is now buried deep within the membrane. Regardless, we recommend exclusive use of synthetic (biocompatible) dialyzers in patients with AKI. The cost differential between synthetic and cellulosic membranes is minimal.
Pore dimensions determine the size selectivity of molecular flux across the membrane. Low flux (small pore) membranes clear small molecules (urea, potassium, and creatinine) but do not clear the larger “middle molecules” which may act as toxins. High flux membranes (large pore) clear middle molecules, such as β2 microglobulin and perhaps inflammatory cytokines generated by AKI and MOSF. This theoretic advantage of high flux membranes may be compromised in settings of low water quality. Large pore size would permit backflow (dialysate to blood) into the patient of endotoxin fragments and other harmful water-borne molecules, such as heavy metals. In settings with high-quality water systems (generally available in the United States), we recommend the use of high flux, large surface area, biocompatible membranes.
Dialysate Composition
Dialysate solutions are composed of specific concentrations of sodium, potassium, bicarbonate, calcium, chloride, glucose, and magnesium. The range and standard concentrations of contents of dialysates for both IHD and PD are summarized in Table 75.3. For IHD, chloride, glucose, and magnesium concentrations are generally fixed. The usual dialysate sodium concentration is 140 mEq per L, but higher concentrations (148 to 150 mEq per L) are often used early in an IHD session to prevent hypotension. Dialysis against a high sodium concentration (148 to 150 mEq per L) results in diffusion of sodium into plasma. This maintains plasma osmolality at a time when urea and other small solutes are being rapidly cleared across the membrane, thus preventing acute intracellular shifts of water that can precipitously lower plasma volume. Later in the dialysis procedure, when urea mobilization is proceeding at a slower pace, dialysate (serum) sodium concentration is returned to normal (140 mEq per L) to prevent hypernatremia. This process of sodium profiling (or sodium modeling) is one of the major strategies employed to prevent hypotension in the AKI patient managed with IHD. Modern dialysis machines offer a variety of sodium modeling profiles. This technique is not needed for CRRT, since solute and volume removal occurs slowly.
Table 75.3 Dialysate Formulation for Hemodialysis and Peritoneal Dialysis | ||||||||||||||||||||||||||||||||||
---|---|---|---|---|---|---|---|---|---|---|---|---|---|---|---|---|---|---|---|---|---|---|---|---|---|---|---|---|---|---|---|---|---|---|
|
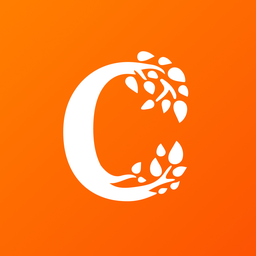
Full access? Get Clinical Tree
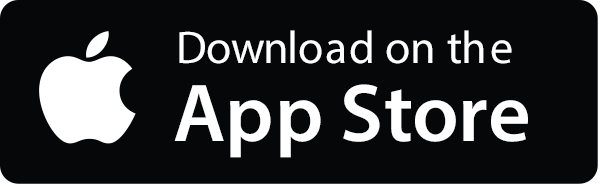
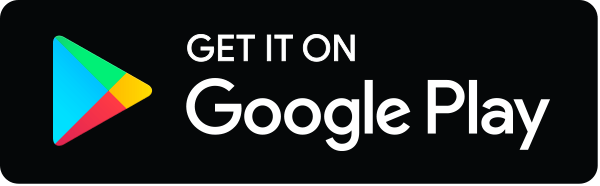