(a) Anatomy and (b) vasculature of the kidney
Blood supply of the kidneys
The kidneys receive 20% of cardiac output via the renal arteries. The renal artery to each kidney divides into two upper branches which supply the anterior and posterior upper poles, and one lower branch which supplies the lower pole. These branches then further divide into vessels called interlobar arteries, arcuate arteries, interlobular arteries and afferent arterioles, which lead to the glomerular capillaries of the nephron (Figure 16.1b).
From the glomerular capillaries, efferent arterioles transport blood to the peritubular capillaries and then to the venous circulation via the interlobular veins, arcuate veins, interlobar veins and renal veins. The transport of blood from glomerular capillaries to peritubular capillaries represents a portal system.
Nerve supply of the kidneys
The innervation of the kidney is supplied by autonomic fibres originating at T10–L1, which reach the kidneys by travelling with the renal vessels. Postganglionic sympathetic fibres are distributed to the afferent and efferent arterioles, the proximal and distal tubules and the juxtaglomerular apparatus. Parasympathetic efferents from the vagus nerve also supply the kidneys, but their function is uncertain. Renal nociceptive afferents enter the spinal cord at T10–11 to give referred pain distributed over the flanks.
The nephron
Each kidney contains approximately a million functional units called nephrons, which are located in the cortex. The nephron consists of a knot of capillaries (the glomerulus) and a tubular system with a blind end. The blind end of the tubular system forms a capsule (Bowman’s capsule) around the glomerulus, which collects the ultrafiltrate from the glomerular capillaries. The other parts of the nephron are the proximal tubule, loop of Henle, distal tubule and collecting duct. The loop of Henle has two limbs, a descending limb and an ascending limb, which are parallel to each other running from the cortex to the renal medulla. Approximately 25% of the nephrons have long loops of Henle, crossing from the cortex deep into the medulla (Figure 16.2).
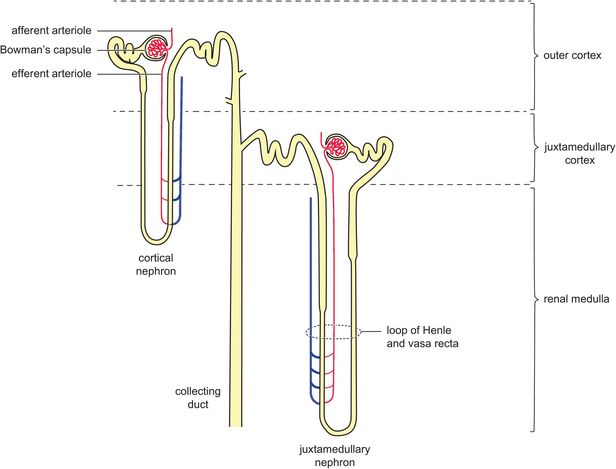
Nephron
The glomerular filter
The function of a glomerulus is to produce an ultrafiltrate of the plasma. It is composed of a knot of capillaries fed by an afferent arteriole and drained by an efferent arteriole (Figure 16.3).
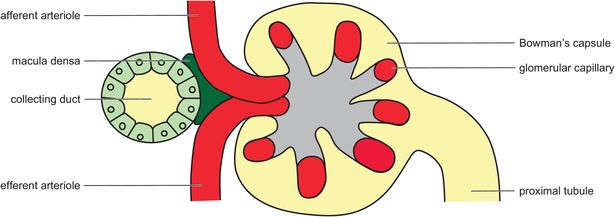
Glomerulus and the juxtaglomerular complex
Mesangial cells are located between the capillaries, and these have a phagocytic and structural role. The glomerular filtration barrier (Figure 16.4) consists of:
Fenestrated capillary endothelium – this layer has many circular fenestrations (pores) between adjacent endothelial cells, with a diameter of about 60 nm. The capillary endothelium acts as a screen to prevent blood cells and platelets from coming into contact with the main filter, which is the basement membrane.
Glomerular basement membrane – this is immediately beneath the glomerular endothelium. It forms a continuous layer, and is the main filtration barrier allowing the passage of molecules according to their size, shape and charge. It consists of collagen and other glycoproteins, including large amounts of heparan sulphate proteoglycan, which is strongly negatively charged.
Visceral epithelial cells of the Bowman’s capsule – these cells are called podocytes and have foot processes (trabeculae) which encircle the basement membrane around the capillary. From the trabeculae, many smaller processes (pedicels) project. Substances pass through the slits (pores) between adjacent pedicels. The podocyte layer also has molecules in the foot processes (integrin, dystroglycan) and slits (nephrin, podocin and CD2-associated protein) which influence its role in maintaining basement membrane integrity and filtration selectivity.
The glomerular ultrafiltrate has a composition echoing that of plasma, which is free from cells and particles of molecular weight > 70,000. Smaller molecules (< 7000) pass freely through the glomerular filter, but permeability to large molecules decreases with size and negative charge.
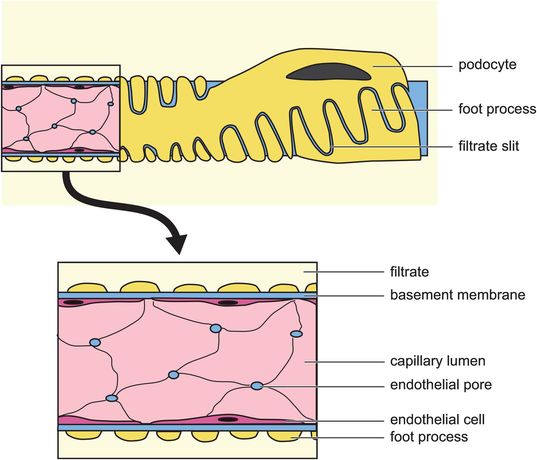
Glomerular filtration barrier
The tubular system
The tubular system of the nephron determines the final composition of the urine. It functions by actively or passively secreting and reabsorbing substances into the glomerular ultrafiltrate.
The tubular system starts at the Bowman’s capsule which surrounds the glomerular capillaries and collects the ultrafiltrate. The ultrafiltrate passes from Bowman’s capsule into the proximal tubule, which feeds into the loop of Henle and from there into the distal tubule. From the distal tubule the filtrate enters the collecting duct system consisting of the cortical and medullary collecting ducts.
It is in the collecting duct system that the degree of water reabsorption or excretion by the kidney is determined and ultimately extracellular fluid osmolarity is maintained.
The majority of nephrons have glomeruli in the outer two-thirds of the cortex and possess short loops of Henle only passing a short distance into the medulla. Approximately 15% of nephrons have glomeruli in the inner third of the cortex (juxtamedullary nephrons), with long loops of Henle passing deeply into the medulla.
The urogenital system
The collecting ducts enter the renal papilla and calyces, in the medulla of each kidney. From here urine drains into the renal pelvis, which is a funnel-shaped expansion of each ureter. Urine is then excreted by travelling in the ureter, bladder and urethra. Normal physiological function may be impaired (i.e. post-renal failure) by anatomical factors:
Renal excretion of waste products
Waste products of metabolism such as urea and creatinine are excreted in urine.
Formation of urine involves:
Filtration of plasma at the glomerulus
Reabsorption of the majority of the filtrate in the tubules
Secretion of additional waste products into the tubules
The rate of urine formation has clinical importance, since it is used in determining the severity of acute kidney injury (Figure 16.5).
Acute kidney injury | ||
Stage | Increase in serum creatinine | Rate of urine output |
1 | of 26.4 μmol L−1by 150–200% | < 0.5 mL kg−1 h−1 for > 6 h |
2 | by 200–300% | < 0.5 mL kg−1 h−1 for > 12 h |
3 | of 354.4 μmol L−1by > 300% | < 0.3 mL kg−1 h−1 for > 24 h0 mL kg−1 h−1 for 12 h |
Chronic kidney disease | ||
Stage | Physiological reduction | Glomerular filtration rate, mL 1.73 cm−2 |
1 | Mostly normal (possible urine dipstick, genetic or structural abnormalities ) | > 90 |
2 | Mild | 60–89 |
3 | Moderate | 30–59 |
4 | Severe | 15–29 |
5 | End-stage | < 15 |
Glomerular filtration
The glomerular filtration rate (GFR) is about 180 litres a day (125 mL min−1). This is equivalent to filtering the total plasma volume every 20 minutes.
Glomerular filtration of molecules and plasma is determined by:
Size of molecules filtered
Charge of the basement membrane
Hydrostatic pressure gradient
Renal plasma flow
Colloid osmotic pressure gradient
Glomerular capillary coefficient
Blood pressure
Size of molecules for filtration
Water and ions are filtered, whereas proteins such as albumin, of molecular weight 69,000, are not normally filtered.
Charge of the glomerular basement membrane
The basement membrane is charged negative, and negatively charged molecules are therefore less readily filtered than those which are positively charged.
The glomerular capillary coefficient (KF)
KF is a measure of the resistance to flow of ultrafiltrate across the total glomerular surface, and is given by the ratio:
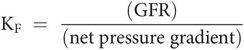
The coefficient has a value of 12.5 mL min−1 mmHg−1. Ultrafiltration across glomerular capillaries occurs approximately 10 times more easily than in other capillary beds.
GFR and colloid osmotic pressure gradient
Changes in filtration fraction will alter colloid osmotic pressure, since ultrafiltration from the glomeruli concentrates the plasma proteins in the glomerular capillaries and increases the colloid osmotic pressure. Any increase in the glomerular colloid osmotic pressure will decrease GFR.
Colloid osmotic pressure gradient is determined by the colloid osmotic pressure in the glomerular capillaries (πG = 32 mmHg), since the colloid osmotic pressure in Bowman’s capsule (πB) is normally zero.
The colloid osmotic pressure in the glomerular capillaries is dependent on the colloid osmotic pressure in the afferent arterioles, the filtration fraction and renal plasma flow.
GFR and renal plasma flow (RPF)
Renal blood flow is 1100 mL min−1, giving a value for renal plasma flow (RPF) of 600 mL min−1. Approximately 20% of renal plasma flow is filtered in the glomeruli giving a value of 120 mL min−1 for GFR. The ratio of glomerular filtration rate (GFR) to renal plasma flow (RPF) is the filtration fraction:
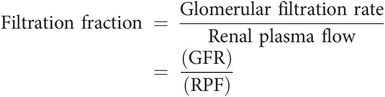
Changes in filtration fraction affect GFR by altering the colloid osmotic pressure (see above).
A decrease in RPF produces an increase in filtration fraction and subsequently a reduction in GFR.
Conversely, an increase in RPF will lead to a decrease in filtration fraction and an increase in GFR.
GFR and hydrostatic pressure gradient
The hydrostatic pressure gradient producing the glomerular ultrafiltrate is equal to the difference between the pressure in the glomerular capillaries (PG = 60 mmHg) and the pressure in Bowman’s capsule (PB = 18 mmHg).
Glomerular hydrostatic pressure is determined by arteriolar resistance at both the afferent and efferent ends of the glomerular capillaries. A reduction in glomerular hydrostatic pressure causes a decrease in GFR. This occurs as a result of factors which increase afferent arteriolar resistance; these include:
An increase in activity of the sympathetic nervous system
Hormones such as noradrenaline, adrenaline and endothelin
An increase in glomerular hydrostatic pressure causes an increase in GFR. This may be caused by factors that:
Decrease afferent arteriolar resistance, e.g. prostaglandins and nitric oxide
Increase efferent arteriolar resistance, e.g. angiotensin II
In addition, an increase in hydrostatic pressure in the Bowman’s capsule reduces the hydrostatic pressure gradient and hence glomerular filtration. Factors which may increase hydrostatic pressure in the Bowman’s capsule include any cause of obstructive renal failure, e.g. renal stones.
The net pressure for glomerular filtration is the difference in hydrostatic pressure gradient and colloid osmotic pressure gradient (Figure 16.6):
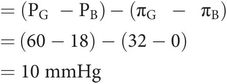
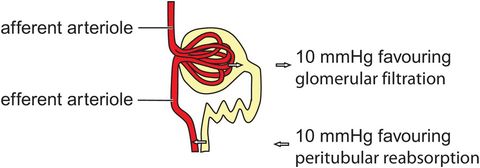
Net filtration pressure in glomerulus and net reabsorption pressure in descending LOH
GFR and blood pressure
GFR remains relatively constant in spite of changes in blood pressure. This process of autoregulation is mediated by:
The myogenic response of blood vessels, which constrict as a result of increases in blood pressure or dilate when blood pressure falls.
The juxtaglomerular complex for tubuloglomerular feedback, which resists changes in glomerular filtration rate.
The juxtaglomerular complex
The juxtaglomerular complex consists of the juxtaglomerular cells in the walls of the afferent and efferent arterioles, and adjacent epithelial cells in the distal tubule called the macula densa (Figure 16.3). Physiological mechanisms involving the juxtaglomerular complex act to prevent a reduction in GFR when blood pressure falls as follows:
A reduction in blood pressure reduces glomerular hydrostatic pressure and initially produces a decrease in GFR.
This decrease in GFR leads to increased absorption of sodium chloride in the loop of Henle, which gives rise to a reduction in the concentration of sodium chloride at the distal tubule and the macula densa.
A release of renin by the macula densa occurs as a result of the reduced sodium chloride concentration, which stimulates the formation of angiotensin I and II.
Angiotensin II leads to preferential vasoconstriction of the efferent arterioles, thus maintaining glomerular hydrostatic pressure and GFR.
In addition, the macula densa causes a reduction in afferent arteriolar resistance, which also acts to maintain glomerular hydrostatic pressure and GFR.
The juxtaglomerular complex provides a feedback mechanism to maintain GFR, by responding to sodium chloride concentration at the distal tubule. It acts by releasing renin, which increases angiotensin II levels.
However, at high angiotensin II levels, GFR is not always maintained because angiotensin II reduces glomerular blood flow, which increases glomerular colloid osmotic pressure and opposes glomerular filtration.
This tubuloglomerular feedback mechanism is driven not only by changes in blood pressure; it is primarily mediated by sodium chloride concentration at the macula densa. For instance, when there is an increase in the delivery of amino acids or glucose to the kidney, a rise in sodium reabsorption in the proximal tubule occurs owing to co-transportation. Under these circumstances tubuloglomerular feedback will also lead to an increase in glomerular hydrostatic pressure and hence in GFR.
Tubular reabsorption and secretion
Glomerular filtrate is processed in three main tubular regions:
The proximal tubule
The loop of Henle
The distal tubule, collecting tubule and collecting duct system
The proximal tubule
Here, effectively 100% of amino acids and 100% of glucose are reabsorbed. 65% of other ions such as sodium, chloride, bicarbonate (via H+ secretion) and potassium are reabsorbed (Figure 16.7), while only about 20% of phosphate filtered is reabsorbed. The normal plasma concentration of urea is 2.5–7.5 mmol L−1, of which 50–60% is secreted. Reabsorption of urea occurs passively down its concentration gradient since the the tubular urea concentration tends to increase as sodium chloride and water are reabsorbed from the proximal tubule. The filtrate osmolarity remains similar to plasma, because 60–70% of the water filtered is also reabsorbed. Organic acids, bases and drugs are secreted into the proximal tubular fluid.
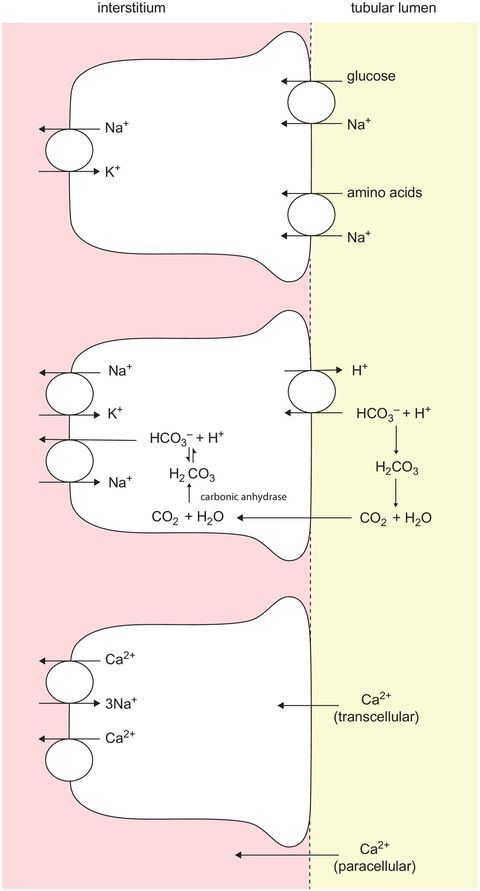
Reabsorption in the proximal tubule
The loop of Henle
The loop of Henle (LOH) is continuous with the proximal tubule and originates in the renal cortex. It consists of a descending limb which passes into the medulla and loops round to become the ascending limb which passes back into the cortex. This limb then continues as the distal tubule. The fluid entering the LOH is initially isotonic compared with plasma, but after traversing the loop, the fluid entering the distal tubule is hypotonic. Thus the tubular fluid is actually diluted during its passage through the LOH. However, the LOH plays a crucial role in the concentration of urine, by functioning as a countercurrent multiplier.
The loops of Henle do not concentrate the tubular fluid within them, but manufacture a hypertonic interstitial fluid in the renal medulla by a countercurrent mechanism. Urine is then concentrated by osmosis from collecting ducts as they pass through the medulla.
The countercurrent mechanism
A proposed mechanism for countercurrent multiplication was described by Wirz, Hargitay and Kuhn in 1951. The hypertonic interstitium in the renal medulla is produced by a small osmotic pressure difference between the ascending and descending limbs of the loops (i.e. a small transverse gradient). This small difference is then multiplied into a large longitudinal gradient by the countercurrent arrangement (i.e. flow in opposite directions) in the two adjacent limbs of the loop.
The ascending limb is not uniform in structure but possesses a thin segment and a thick segment. This limb produces an increase in the surrounding interstitium by the extrusion of sodium and accompanying ions. Only the thick segment actively extrudes ions. Here 25% of ions such as sodium, chloride, bicarbonate (via H+ secretion) and potassium are reabsorbed, and 65% of magnesium is reabsorbed (Figure 16.8).
Tubular fluid becomes hypo-osmolar because both thin and thick segments are impermeable to water, so that water is unable osmotically to follow the extruded ions. Consequently, the osmolality of the medullary interstitium is increased and the osmolality of the fluid in the ascending limb is decreased.
The descending limb is permeable to water and, to a lesser extent, is also permeable to NaCl. The fluid within the descending limb will therefore come to osmotic equilibrium with the interstitium. In effect, then, we can consider the transport of NaCl out of the ascending limb as being directed into the descending limb.
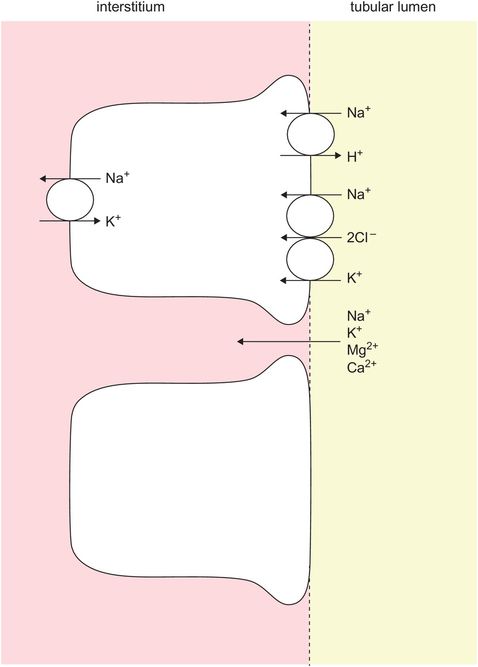
Extrusion in the thick ascending loop of Henle
Long and short loops of Henle
Only 25% of the nephrons (the juxtamedullary nephrons) have long loops of Henle which pass deeply into the medulla. The remaining 75% of nephrons (cortical nephrons) have short loops of Henle which barely reach the medulla, and these nephrons do not make a significant contribution to the manufacture of medullary hypertonicity. However, the collecting tubules of all the nephrons (both cortical and juxtamedullary) pass through the medulla. Thus only the long-looped nephrons, which form 25% of the total, produce the medullary gradient that concentrates the urine produced by all the nephrons.
The distal tubule
The ‘distal tubule’, although anatomically defined, is not a distinct section of the nephron in terms of physiological function. Functionally the distal tubule is a segment of the nephron in which the tubule cells undergo transition from ascending-limb-of-Henle-type cells to collecting-tubule-type cells.
The walls of the ascending-limb-of-Henle-type cells have only a very low and essentially constant permeability to water. On the other hand the collecting-tubule-type cells have a variable water permeability, which is regulated by the hormone ADH (antidiuretic hormone, vasopressin).
The collecting tubules have cortical and medullary sections, each section having somewhat different properties. Both are relatively impermeable to water, urea and NaCl, but the water permeability is increased by ADH. Thus, ADH leads to urine concentration by permitting the osmotic abstraction of water into the interstitium.
In the distal tubule and collecting tubules and ducts, variable percentages of sodium, chloride and bicarbonate (via H+ secretion) may be reabsorbed (Figure 16.9). The osmolarity of the tubular fluid is controlled, and may become high if there is increased water reabsorption and low if there is increased water clearance.
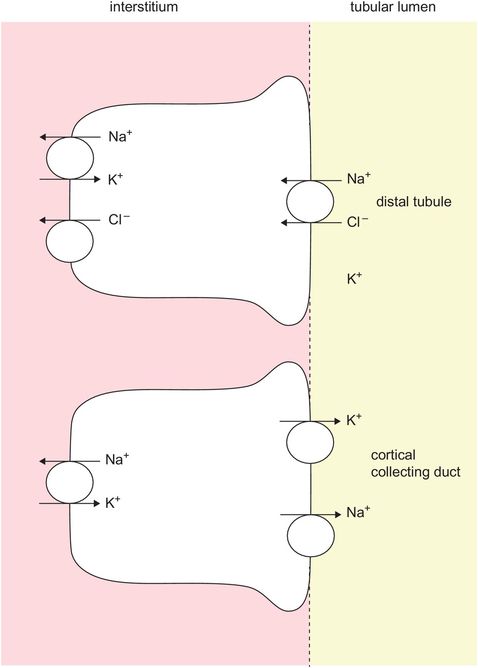
Reabsorption in distal tubule and collecting duct cells
Tubular transport
Transport of substances, ions and water takes place between the tubular filtrate and the peritubular capillaries. The transport path traverses the tubular cells, passing into the interstitium or lateral intercellular spaces (LIS), and from there into the peritubular capillaries. Transport occurs via the following routes:
Across the tubular cells, via its luminal and basolateral sides (transcellular pathway) and into the LIS
Between luminal cells (paracellular pathway) into the LIS
From the LIS across the capillary membrane into the peritubular capillaries
The general mechanisms are the same in both reabsorption and secretion. The difference between reabsorption and secretion lies in the direction of transport.
General mechanisms of transport
Active transport, an energy-dependent process involving Na+/K+ATPase on the basolateral side of the tubular cell. Sodium is actively pumped out of the tubular cell into the interstitium, thus lowering its concentration and creating a negative charge. Other pumps include H+ATPase and Ca2+ATPase.
Facilitated diffusion of sodium, a process in which sodium is transported from the tubular lumen into the tubular cell, on a carrier protein, along its electrochemical gradient.
Co-transport of sodium with other substances, e.g. glucose, amino acids, hydrogen, chloride and potassium ions, on carrier proteins. These substances undergo secondary active transport as they move against a concentration gradient.
Reabsorption of water by osmosis.
Water reabsorption
There is no active water reabsorption. Water reabsorption in the nephron follows solute absorption and can be both transcellular and paracellular.
It has become clear in recent years that much of the water movement through epithelia is transcellular, and occurs via specific water channel proteins, termed aquaporins (AQP). Several different aquaporins have been identified:
AQP1 (also called CHIP-28, or 28-kilodalton channel-forming integral protein) is abundant in the apical and basal membranes of renal proximal tubule cells, and the cells of the thin descending limb of Henle, as well as in many extrarenal tissues.
AQP2 is the vasopressin (ADH)-sensitive channel, discussed in more detail later (see Figure 16.11).
AQP3 is in the basolateral membranes of the collecting duct principal cells, where it provides an exit route for water.
AQP4 is abundant in the brain, and may be important in the hypothalamic osmoreceptor cells.
Rates of tubular reabsorption
Transport mechanisms for some substances and in some parts of the tubular system may become saturated, resulting in a maximum reabsorption rate called the transport maximum. An example is the transport maximum for glucose in the proximal tubule in diabetic patients, when the filtered glucose cannot be reabsorbed completely and an excess appears in the urine. Similarly there is a maximum value for the reabsorption of sodium in the distal tubule.
Alternatively a maximum value for reabsorption may not occur, as in the case of sodium transport in the proximal tubule. This transport mechanism does not appear to reach saturation, but continues to increase as the sodium load increases. The transport mechanism here is dependent on the electrochemical gradient of sodium and time.
The maximum reabsorption rate of some substances, such as Na+, is also affected by leakage from the LIS back into the tubular lumen (or ‘backflux’). This in turn is affected by the rate of peritubular capillary uptake.
Peritubular capillary reabsorption
Reabsorbed fluid returns to the circulation by uptake of fluid from the LIS into the peritubular capillaries (Figure 16.6). The overall reabsorption rate is 124 mL min−1, just less than the glomerular filtration rate of 125 mL min−1.
Peritubular capillary uptake is dependent on:
Colloid osmotic pressure gradient of 17 mmHg, arising from the difference between 32 mmHg in the peritubular capillary and 15 mmHg in the LIS fluid. This pressure gradient favours reabsorption into the capillaries.
Peritubular hydrostatic pressure gradient of 7 mmHg, arising from the difference between 13 mmHg in the peritubular capillary and 6 mmHg in the LIS. This pressure gradient resists fluid reabsorption into the capillaries.
Filtration coefficient (KF = 12.4 mL min−1 mmHg−1) for the peritubular capillaries, determined by the product of the permeability of the capillary walls and their total surface area.
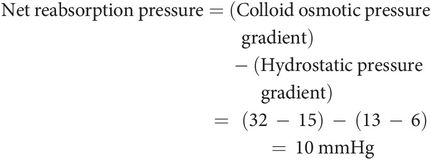
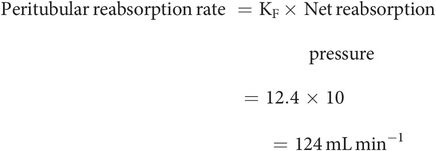
These pressure gradients explain the phenomenon of glomerulotubular balance, a process in which a rise in glomerular filtration and hence tubular load leads to increased peritubular capillary reabsorption. This physiological mechanism occurs because:
A rise in glomerular filtration rate or a reduction in plasma flow, e.g. due to angiotensin II, leads to an increase in filtration fraction.
The increase in filtration fraction causes an increase in peritubular colloid osmotic pressure, which favours reabsorption.
This process acts to ensure that the distal tubule, cortical collecting tubule and medullary collecting duct receive a relatively constant volume of filtrate. However, this mechanism is attenuated by high blood pressure, which leads to an increase in peritubular capillary hydrostatic pressure, which decreases peritubular reabsorption.
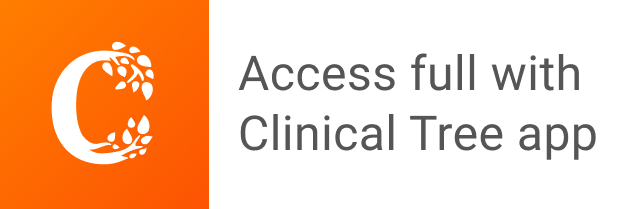