The Glomerulus
Structure and Function of the Glomerulus
Glomeruli are found in the renal cortex and consist of a tuft of capillaries surrounded by Bowman’s capsule, the dilated blind end of the renal tubule. Glomerular capillaries are uniquely interposed between two sets of arterioles. Blood flows from the afferent arterioles through the glomerular capillaries and then on to the efferent arterioles. Hence, pressure in the glomerular capillaries is a function of the vascular activity of both the afferent and efferent arterioles (Fig. 16-2): Afferent vasoconstriction lowers glomerular capillary pressure, whereas efferent arteriolar vasoconstriction raises glomerular capillary pressure. Glomerular capillary pressure causes water and low-molecular-weight substances to be filtered into Bowman’s capsule and the renal tubule system.

Fluid that is filtered from the glomerular capillaries into the renal tubules is called glomerular filtrate. Because the glomerular capillary membrane contains pores, its permeability is much greater than that of the typical tissue capillary. Fluid, amino acids, and ions are rapidly filtered, whereas higher molecular weight proteins are retained within the capillary. The composition of glomerular filtrate, then, is similar to plasma but without protein.
Glomerular Filtration Rate
The volume of collective glomerular filtrate formed over time is called the glomerular filtration rate (GFR). In a normal person, the GFR is approximately 125 mL per minute or 180 L per day. Because 99% of this 180 L of glomerular filtrate is reabsorbed, daily urine output is 1 to 2 L. As described earlier, glomerular capillary pressure leads to filtration into the renal tubule. The normal filtration pressure of approximately 10 mmHg is calculated as glomerular capillary pressure (60 mm Hg) minus colloid osmotic pressure (32 mm Hg) and pressure in Bowman’s capsule (18 mm Hg) (Fig. 16-3). The filtration rate is influenced by several factors: Mean arterial pressure, cardiac output, and the sympathetic nervous system may each raise glomerular capillary pressure and increase the GFR.

The kidneys have an autoregulatory mechanism to modulate the effect of mean arterial pressure on the GFR. This process of autoregulation involves feedback from the distal renal tubules to the glomerulus. Specialized cells in the distal renal tubules, called the macula densa, signal the afferent or efferent renal arterioles to either vasoconstrict or vasodilate. Through the consequent adjustment of glomerular capillary pressure, a nearly constant filtration pressure leads to a consistent GFR across a range of mean arterial pressure, remaining relatively constant between mean arterial pressures of 60 and 160 mm Hg. Because even a small change in GFR can lead to wide variations in urine output, it is clear that tubule–glomerular feedback serves an important role in homeostasis.
The Renal Tubule
Structure of the Renal Tubule
The renal tubule is composed of the proximal convoluted tubule, the loop of Henle, and the distal convoluted tubule1 (see Fig. 16-1). As described earlier, the loops of Henle in juxtamedullary nephrons extend into the renal medulla before returning filtrate back to the renal cortex in the distal convoluted tubule. Glomerular filtrate, after passing along the length of the renal tubule, then enters the collecting duct, which is a confluence of flow from several nephrons into the renal pelvis.
Renal Tubular Function
Glomerular filtrate is converted into urine along the course of the renal tubule2 (Table 16-1). The majority of water and various solutes that are filtered by glomerular capillaries are reabsorbed into peritubular capillaries. Metabolic waste products, also filtered by glomerular capillaries, are not reabsorbed. Other solutes are secreted by renal tubular epithelial cells into the lumen of the renal tubule. Thus, the urine found in the collecting duct is composed mainly of substances filtered through the glomerular capillaries and a small amount of secreted substances. The process of reabsorption determines the volume of urine formed, whereas secretion is particularly important in determining the nature of the urine, such as concentration of potassium and hydrogen ions. Various portions of the renal tubule have differing roles in reabsorption and secretion2 (see Table 16-1). Approximately two-thirds of all reabsorption and secretion in the renal tubules occurs in the proximal renal tubules. The most important factors influencing the reabsorption of sodium and water are aldosterone, arginine vasopressin (AVP), renal prostaglandins, and atrial natriuretic peptide.

Reabsorption of sodium involves moving this ion against a concentration gradient from the lumen of the proximal tubule into peritubular capillaries. This process requires energy, supplied by the sodium–potassium adenosine triphosphatase (ATPase) system and is appropriately called active transport. Other transport processes along the tubule, including glucose reabsorption, amino acid reabsorption, and organic acid secretion, share a common carrier with sodium. Hence, the great majority of transport across renal tubular cells is dependent on sodium–potassium ATPase activity. The proximal convoluted renal tubules, driving the ATPase enzyme for sodium reabsorption, consume approximately 80% of renal oxygen consumption.3
More than 99% of the water in the glomerular filtrate is reabsorbed into peritubular capillaries as it passes through renal tubules. The variation in permeability of epithelial cells lining the tubules is important in renal function. Rapid osmosis of water through proximal renal tubules means that the concentration of solutes on the capillary side of cell membranes is almost never more than a few milliosmoles greater than in the tubular lumen. However, the distal tubules are almost completely impermeable to water, allowing for control of the specific gravity of the urine. The permeability of the collecting ducts is variable and determined by the action of AVP. When AVP activates adenylate cyclase in the epithelial cells lining the collecting duct, the resulting cyclic adenosine monophosphate increases permeability of cell membranes to water. Hence, increased AVP leads to reabsorption of water from the collecting ducts, resulting in highly concentrated urine. Decreased AVP results in little water reabsorption and large amounts of dilute urine.
Countercurrent System
The ability of the kidneys to produce either dilute or concentrated urine depends on the gradient in osmolarity between the renal cortex and renal medulla that is created by the loop of Henle. Whereas the renal cortex has a relatively low osmolarity (300 mOsm/L), the renal medulla contains highly concentrated interstitial fluid (1,400 mOsm/L near the renal pelvis) due to active reabsorption of solutes in the loop of Henle3 (Fig. 16-4). The high medullary osmolarity is maintained in part by sluggish blood flow, preventing the removal of solutes.

Just as the juxtamedullary loops of Henle carry glomerular filtrate from the cortex into the renal medulla and back to the cortex, the vascular supply has a similar structure. The U-shaped arrangement of peritubular capillaries, known as the vasa recta, parallels the loops of Henle. This forms a countercurrent system, in which capillary inflow runs parallel and in an opposite direction to capillary outflow.
Aquaporins
The high osmolarity in the renal medulla allows for the potential for quick reabsorption of water by osmosis as filtrate passes through the renal collecting ducts. This process is mediated by aquaporins, which are channels that facilitate rapid passage of water across lipid cell membranes at a velocity greater than possible by simple diffusion.4–6 They are tetramer protein structures and are found in the kidneys, brain, salivary and lacrimal glands, and respiratory tract. Five aquaporins in the kidney have a role in water balance.5 Aquaporin-1 is in the proximal renal tubules, while aquaporin-2 is found in the renal collecting ducts. In response to AVP, the channels in the tubular collecting duct edpithelium are opened, leading to reabsorption of water and formation of concentrated urine. In the absence of AVP, dilute urine traverses the collecting ducts without being affected by medullary osmolarity.
Tubular Transport Maximum
Tubular transport maximum (Tmax or Tmax) is the maximum amount of a substance that can be actively reabsorbed from the lumens of renal tubules each minute. The Tm depends on the amounts of carrier substance and enzyme available to the specific active transport system in the lining epithelial cells of renal tubules.
The Tmax for glucose is approximately 220 mg per minute. When the amount of glucose that filters through the glomerular capillary exceeds this amount, the excess glucose cannot be reabsorbed and passes into urine (Fig. 16-5). The usual amount of glucose in the glomerular filtrate entering proximal renal tubules is 125 mg per minute, and there is no detectable loss into urine. When the tubular load, however, exceeds approximately 220 mg per minute (threshold concentration), glucose begins to appear in urine. A blood glucose concentration of 180 mg/dL in the presence of a normal GFR results in delivery of 220 mg per minute of glucose into the renal tubular fluid. Loss of glucose in urine occurs at concentrations above the Tm for glucose. The presence of large amounts of unreabsorbed solutes in the urine such as glucose (or mannitol) produces osmotic diuresis by retaining water in the collecting system.

Transport of Urine to the Bladder
From the collecting ducts, urine travels into the renal pelvis. A ureter arises from the pelvis of each kidney. At its distal end, the ureter penetrates the bladder obliquely such that pressure in the bladder compresses the ureter, thereby preventing reflux of urine into the ureter when bladder pressure increases during micturition.
Each ureter is innervated by the sympathetic and parasympathetic nervous system. As urine collects in the renal pelvis, the pressure in the pelvis increases and initiates a peristaltic contraction that travels downward along the ureter to force urine toward the bladder. Parasympathetic nervous system stimulation increases the frequency of peristalsis, whereas sympathetic nervous system stimulation decreases peristalsis.
Obstruction of a ureter by a stone causes intense reflex constriction and pain. In addition, pain elicits a sympathetic nervous system reflex (ureterorenal reflex) that causes vasoconstriction of the renal arterioles and a concomitant decrease in urine formation in the kidney when there is obstruction of the ureter.
As the bladder fills with urine, stretch receptors in the bladder wall initiate micturition contractions. Sensory signals are conducted to the sacral segments of the spinal cord through the pelvic nerves and then back again to the bladder through parasympathetic nervous system fibers. The micturition reflex is a completely automatic spinal cord reflex that can be inhibited (tonic contraction of the external urinary sphincter) or facilitated by centers in the brain. Spinal cord damage above the sacral region leaves the micturition reflex intact but is no longer controlled by the brain.
Renal Blood Flow
Although the kidneys represent about 0.5% of total body weight, their blood flow is disproportionately large at 20% to 25% of the cardiac output.3 Renal blood flow is approximately 400 mL/100 g/minute, compared with 70 mL/100 g/minute for the heart and liver. The ability to autoregulate keeps renal blood flow relatively constant across a range of systemic mean arterial pressures. Because renal blood flow is large, the fraction of oxygen extraction is low despite high oxygen consumption, the PO2 decreasing from 95 mm Hg in the renal artery only to about 70 mm Hg in the renal vein.
Approximately 90% of the renal blood flow is distributed to the renal cortex, with less than 10% of renal blood flow going to the medulla. The generous delivery of blood to the cortex supports flow-dependent functions such as glomerular filtration and tubular reabsorption processes of the cortex. By contrast, low blood flow in the medulla maintains a high interstitial fluid osmolarity, which in turn permits concentration of the urine.7 Low blood flow also makes the medulla more susceptible to ischemia than the cortex.
Renal Cortex Blood Flow: Glomerular and Peritubular Capillaries
Blood enters the renal artery, whose branches ultimately supply the afferent arterioles of the glomeruli. As described earlier, afferent arterioles feed the glomerular capillary bed, out of which blood flows into the efferent arterioles1 (see Fig. 16-1). The vascular resistance of the efferent arterioles raises glomerular capillary pressure, which promotes continuous filtration of fluid from glomerular capillaries into Bowman’s capsule. Blood flows from the arterioles into a second capillary network called peritubular capillaries. These capillaries have substantially lower pressure8 (see Fig. 16-3), promoting reabsorption of fluid from the tubules into the peritubular capillaries.
Renal Medulla Blood Flow: The Vasa Recta
Capillaries that descend with the loops of Henle are referred to as the vasa recta, which receive only 1% to 2% of renal blood flow. These capillaries, after descending into the renal medulla, return to the renal cortex and empty into veins. As described earlier, this countercurrent system minimizes the washout of solutes from the interstitial fluid of the medulla creating a high osmolarity that promotes the absorption of water from the collecting ducts and the formation of concentrated urine.
Autoregulation of Renal Blood Flow
Renal blood flow and GFR are kept relatively constant within a range of mean arterial pressure between approximately 60 and 160 mm Hg8 (Fig. 16-6). Because the GFR parallels renal blood flow, cardiac output has an important effect on the GFR. The mechanism for autoregulation is controversial.9 One theory is a myogenic response, whereby increased perfusion pressure leads to an increased wall tension in the afferent arterioles, resulting in automatic contraction of the smooth muscle fibers in the vessel wall. This increases resistance keeping flow constant despite the increase in perfusion pressure.3 An alternative hypothesis is that a tubuloglomerular feedback mechanism is responsible for autoregulation, whereby increased perfusion pressure will increase filtration, increasing the tubular fluid delivery to the macula densa, which then releases a factor or factors that cause vasoconstriction.

In the setting of decreased effective circulating volume, however, renal blood flow may be decreased despite adequate perfusion pressure. Activation of the sympathetic nervous system shunts cardiac output away from the kidneys. Hence, adequate systemic blood pressure does not necessarily indicate adequate renal perfusion in the presence of hypovolemia.
Juxtaglomerular Apparatus
The juxtaglomerular apparatus is where the distal renal tubule passes between the afferent and efferent arterioles. Epithelial cells of the distal renal tubules that contact these arterioles are called the macula densa, whereas corresponding cells in the arterioles are known as juxtaglomerular cells. In response to decreased renal blood flow, juxtaglomerular cells release renin into the circulation3 (Fig. 16-7). Renin converts angiotensinogen to angiotensin I, which is then converted to angiotensin II by angiotensin-converting enzyme. Effects of angiotensin II include thirst, vasoconstriction, and salt and water reabsorption by the kidneys to maintain circulating volume and increase renal blood flow. Whether the initial cause of decreased renal blood flow is the result of hypovolemia, systemic hypotension, or sympathetic nervous system stimulation, the effect of renin is to maintain renal blood flow and GFR.

Regulation of Body Fluid
The kidneys have a primary role in the regulation of the amount and nature of body fluids. They control the following characteristics:
• Blood and extracellular fluid volume
• Osmolarity of body fluids
• Plasma concentration of ions and urea
Blood and Extracellular Fluid Volume
Blood volume is maintained over a narrow range despite large daily variations in fluid and solute intake or loss. The mechanism for control of blood volume also affects systemic blood pressure and cardiac output, as these are interrelated. An increase in blood volume increases the cardiac output, which usually increases the systemic blood pressure. Increased cardiac output and systemic arterial pressure will increase renal blood flow and GFR, resulting in an increase in urine output. The negative feedback loop is completed by a consequent decrease in circulating blood volume.
This basic regulatory mechanism is augmented by other factors. In the setting of decreased blood volume, an increased circulating concentration of AVP will increase water reabsorption, whereas an increase in aldosterone promotes sodium reabsorption and thus an osmotic reabsorption of water. This decreases urine volume and restores blood volume. Another factor is mediated through atrial stretch receptors and atrial natriuretic peptide to be described in the next section.
Regulation of normal circulating blood volume is impaired by factors directly affecting vascular capacitance. Persistent vasoconstriction associated with essential hypertension or sympathetic nervous system stimulation (from pheochromocytoma, for example) results in a decrease in blood volume. Conversely, blood volume may be increased by chronic drug-induced vasodilation or the effects of severe varicose veins.
The regulation of extracellular fluid volume is controlled indirectly through the maintenance of circulating blood volume. An increase in blood volume leads to an increase in extracellular fluid volume, whereas decreased extracellular fluid volume accompanies reduced blood volume. Although these volumes move in the same direction, their proportional change is affected by capillary permeability, which is commonly influenced by perioperative factors. The extracellular fluid space may be considered as a reservoir for excess intravenous fluid administered during the perioperative period.
Atrial and Renal Natriuretic Factors
Cardiac atrial muscle synthesizes and secretes a peptide hormone known as atrial natriuretic peptide (ANP), which is released in response to increased right and left atrial pressure and volume. ANP binds to receptors in the renal collecting ducts and, acting via transcellular second messenger systems, inhibits sodium reabsorption. Hence, atrial “stretch” promotes elimination of sodium and water, and a subsequent decrease in circulating volume. ANP additionally has vasodilatory properties, thereby lowering systemic blood pressure and eliciting renal artery vasodilation.
The renal analogue of ANP is renal natriuretic peptide (urodilatin), which is synthesized in renal cortical nephrons. It is likely that ANP is primarily a cardiovascular regulator and relatively unimportant for sodium excretion, whereas renal natriuretic peptide participates in the intrarenal regulation of sodium excretion.3,10 In mechanical ventilation, positive end-expiratory pressure reduces atrial distension and atrial transmural pressure. This reduces ANP release, which may contribute to sodium and water retention by the kidneys.
Osmolarity of Body Fluids
The primary determinant of body fluid osmolarity is the concentration of sodium in the extracellular fluid. Sodium ion concentration is largely controlled by two mechanisms: the osmoreceptor–AVP response and the thirst reflex. Aldosterone, by contrast, has a minimal role in the maintenance of sodium concentration and plasma osmolarity8 (Fig. 16-8). Aldosterone-induced reabsorption of sodium is accompanied by reabsorption of water. For this reason, patients with primary hyperaldosteronism typically have increased extracellular fluid volume but nearly normal serum sodium levels.

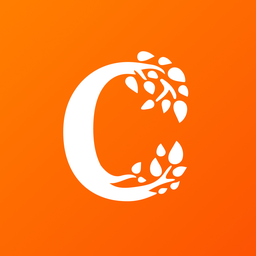
Full access? Get Clinical Tree
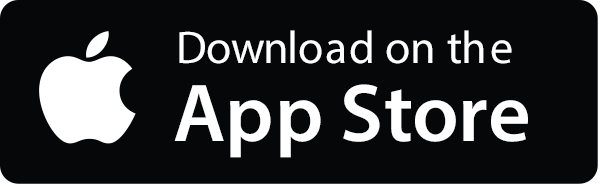
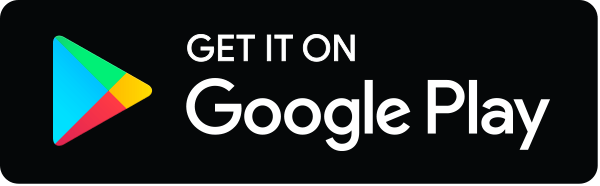