Jamie L. Sparling, Marcos F. Vidal Melo The practice of thoracic anesthesia involves not only patients with impaired pulmonary function, it also necessitates dynamic changes in ventilation with resulting impact on perfusion and ventilation/perfusion matching. Thus it is imperative that the thoracic anesthesiologist has a thorough understanding of the normal pulmonary physiology, as well as states of pathophysiology. Further, he or she must have an intimate familiarity with the impact of mechanical ventilation on both normal and injured lungs, so he or she is equipped to manipulate ventilation in a way that responds to the dynamic needs of thoracic surgery, as well as the evolution of lung injury. Lung mechanics is the expression of lung function through measures of pressure and flow. In this chapter, we review normal lung structure and physiology, an overview of lung mechanics, clinical testing of lung function, and the implications and effects of anesthetics on these factors. lung mechanics; pulmonary function testing; compliance; transpulmonary pressure; driving pressure; esophageal pressure The practice of thoracic anesthesia, in addition to involving patients with impaired pulmonary function, also necessitates dynamic changes in ventilation that impact perfusion and ventilation/perfusion matching. Thus it is imperative that the thoracic anesthesiologist has a thorough understanding of normal pulmonary physiology, as well as states of pathophysiology. Further, the practitioner must have an intimate familiarity with the impact of mechanical ventilation on both normal and injured lungs, to be able to manipulate the ventilation in response to the dynamic needs of thoracic surgery, as well as the evolution of lung injury. Lung mechanics is the expression of lung function through measures of pressure and flow.1 In this chapter, we review normal lung structure and physiology, an overview of lung mechanics, clinical testing of lung function, and the implications and effects of anesthetics on these factors. The trachea extends from the cricoid cartilage at the level of C6 to the carina at approximately the level of T4/5, at which point it branches into the right and left mainstem bronchi. The trachea is approximately 12 cm in women and 14 cm in men, and ranges from a mean of 19 ± 1.5 mm in diameter in women to 22 ± 1.5 mm in men. The right mainstem bronchus is shorter, wider, and more vertical than the left mainstem bronchus. Its length is approximately 1.4 to 1.8 cm compared with 4.4 to 4.9 cm on the left; thus the right lung is generally more susceptible to aspiration. There are three right-sided lobes: the upper, middle, and lower lobes. On the left, there are two lobes, the upper and lower lobes, with the lingula being a “tongue-like” extension of the left upper lobe. The lobes are covered with visceral pleura and are separated by folds of pleura known as the horizontal and oblique fissures on the right, and by the oblique fissure on the left. Each lobe has a lobar, or secondary bronchus, as well as lobar blood supply. The lobes are further divided into bronchopulmonary segments, which again have dedicated named bronchi and blood supply. The right and left lungs each have 10 segments, although nomenclature for these segments may vary slightly in the literature (Fig. 5.1).2,3 The primary, or mainstem, bronchi branch from the trachea at the carina, then further branch into the lobar bronchi, and the segmental bronchi. These airways contain rings of cartilage that help keep them open. Once the airways branch into bronchioles, the walls are made of smooth muscle, and at the level of the respiratory bronchioles, the walls are made of only epithelium. This marks the transition from the conducting airways, encompassing the oropharynx and nasopharynx through bronchioles, to the respiratory airways, which participate in gas exchange and include the respiratory bronchioles and the alveoli.2 On average, the lungs have 23 generations of dichotomous branching, as shown in Fig. 5.2.4 The respiratory bronchioles end in the acinus, which is the functional unit of the lung, and consists of the alveolar ducts and alveolar sacs. The acinus appears as a cluster of grapes, each of which may contain up to 2000 alveoli. In total, the human lung consists of approximately 300 million alveoli creating a surface area for gas exchange of 40 to 80 m.2,4 The alveoli contain alveolar type I cells, alveolar type II cells, and alveolar macrophages. Type I cells are squamous cells, comprising the very thin alveolar walls to facilitate gas exchange, and they cover up to 95% of the surface area of the alveoli. Type II cells are cuboidal and contain lamellar bodies, which secrete pulmonary surfactant, a film of phospholipids which reduces alveolar surface tension to facilitate lung expansion. Alveolar macrophages are scavengers that engulf foreign particles throughout the lungs, playing a key immunologic role.2,3 Lung volume at any given point in time depends upon lung and chest wall mechanics, the activity of the diaphragm and accessory muscles of respiration, and the size of the individual, as well as the ambient conditions (temperature, pressure, humidity) in which such volumes are measured. The standard lung volumes and capacities measured by spirometry are expressed at body temperature, atmospheric pressure, saturated with water vapor (BTPS), and are compared with volumes predicted by the individual’s age, sex, and body size.5 Measures of lung volume are denoted as either “volumes” or “capacities,” where volumes are not subdivided, whereas capacities are the sum of two or more standard lung volumes. Of note, lung volumes correlate better with impairment of patient functional capabilities than respiratory function measurements related to airflow.6 The tidal volume (VT) is the volume of gas inhaled and exhaled with each breath. With quiet breathing at rest, it is approximately 500 mL per breath for a 70-kg adult, that is, 5 to 7 mL/kg of predicted body weight. The VT may be augmented considerably in several conditions (e.g., during exercise) and is achieved by increased work of the diaphragm and the accessory muscles of respiration.5 The amount of gas left in the lungs following a forced maximal exhalation is known as the residual volume (RV). It is determined by the balance between the outward expansion of the chest wall opposing the inward recoil of the lungs with the force generated by the muscles of expiration. Normal RV for a 70-kg adult is around 1.5 L, but because of dynamic airway collapse with forced expiratory effort, gas may become trapped in the alveoli. Thus the RV can become much larger in emphysema, where the lungs’ inward recoil is diminished and amount of gas trapping increases.5 The expiratory reserve volume (ERV) is the difference between the functional residual capacity (FRC) and the RV, or the amount of gas that can be forcibly exhaled at the end of a normal tidal exhalation. Similarly, the inspiratory reserve volume (IRV) is the volume of gas that can be forcibly inhaled starting at the end of a normal tidal inhalation. The ERV is approximately 1.5 L, while the IRV is approximately 2.5 L in a healthy 70-kg adult.5 FRC is the volume of gas remaining in the lungs at the end of a normal tidal exhalation; therefore it is the sum of the RV and the ERV. It is determined by the balance of the inward recoil of the lungs and the outward expansion of the chest wall, as well as the level of tone of the respiratory muscles. The FRC is approximately 3 L in a healthy 70-kg adult.5 The point at which the inward recoil is exactly balanced by the outward expansion is known as the relaxation volume (Vr). FRC may be equal to, greater than, or less than the Vr, depending on the circumstances. For instance, in obstructive lung disease, relaxed exhalation can be incomplete as the next inspiratory effort starts resulting in FRC greater than Vr.7 Lung hyperinflation results in increased RV and FRC. Static hyperinflation is produced by the reduction in lung elasticity because of emphysema. It can also be caused by excessive positive end-expiratory pressure (PEEP). Dynamic hyperinflation results from air trapping from incomplete exhalation of inhaled gas. Interestingly, during mechanical ventilation, while PEEP adds to the risk of hyperinflation in chronic obstructive pulmonary disease (COPD) patients, it can also result in reduced hyperinflation in a subset of COPD patients because of airways being “stented open” by its application.8 The total lung capacity (TLC) is the maximal volume of gas in the lungs after a maximal inhalation; thus it is the sum of the RV, ERV, VT, and IRV. TLC is approximately 6 L for a healthy 70-kg adult. The vital capacity (VC) is the maximal volume of gas exhaled during a forced exhalation after a forced inhalation. Thus VC is the sum of the VT, IRV, and ERV. The VC is approximately 4.5 L in a healthy 70-kg adult.5 Fig. 5.3 shows a summary of how the standard lung volumes and capacities relate, along with average values for a 70-kg adult. Closing capacity (CC) is the lung volume at which small airway closure begins to occur. CC is the sum of the closing volume (CV) and RV.9 Usually, the CC is smaller than FRC, so airways do not close with normal tidal breathing. However, age,10 supine positioning, pregnancy,11 and obesity,12 among other conditions, all increase CC. Airway closure at a larger CC increases the likelihood of hypoxemia. CV is measured using the nitrogen washout test, as illustrated in Fig. 5.4.13 In this method, the subject takes a breath of 100% oxygen and exhales through a one-way valve measuring nitrogen content and volume of the gas exhaled. The initial portion of the curve where the nitrogen content is zero is the dead space, where no gas exchange has occurred. The nitrogen content increases as the patient breathes a VC breath to RV. The CV is the volume between the terminal point of slope change and the RV. CC is the sum of the CV measured and the RV.14 As CC increases with age, CC exceeds FRC, resulting in small airway closure during normal tidal breathing. This transition occurs at a younger age in the supine patient (approximately 44 years) compared with the upright patient (approximately 66 years), whereas FRC does not change with age (Fig. 5.5). It is well accepted that supine positioning and subsequent induction of general anesthesia with neuromuscular blockade reduces FRC, with reports of as much as a 9% decrease.15 This reduction of FRC occurs consistently following induction, and progresses at a slower rate throughout the duration of anesthesia; it is further accentuated in obese patients.16 The sitting position, as compared with supine, invariably increases FRC, even in patients under general anesthesia, as it favors the caudad displacement of the diaphragm.17 Importantly, recruitment breaths and increased VT may temporarily recruit consolidated lung volume under anesthesia, but they are insufficient to keep an atelectatic lung open. This concept has been demonstrated both experimentally and in humans. In a study of pigs undergoing one lung ventilation, the ventilated lung experienced substantial V./Q. mismatch, hyperperfusion, and alveolar damage on histologic examination.18 Further, tidal recruitment with a VT of 10 mL/kg increased the volume of the ventilated lung that was normally aerated (versus consolidated), but applied cyclic recruitment was associated with the initiation of an injurious immune response.19,20 Similarly, in a rabbit model, one lung ventilation with high VTs and zero PEEP resulted in higher mean pulmonary artery pressure (MPAP), greater lung weight, and higher levels of thromboxane B2, all suggestive of acute lung injury (ALI).21 In morbidly obese patients, the effect of increased PEEP alone, a recruitment maneuver alone, and a recruitment maneuver followed by increased PEEP were compared using computed tomography (CT) scans and arterial partial pressure of oxygen (PaO2)/inspired oxygen fraction (FiO2) ratios. Similar to animal studies, the recruitment maneuver only transiently improved respiratory function, and PEEP by itself could not recruit the lungs, whereas the combination of the recruitment maneuver with the increased PEEP improved PaO2/FiO2 ratios and reduced atelectasis on CT.22 The transpulmonary pressure, PL, represents the distending pressure across the lungs, which is the difference between the airway pressure and the pleural pressure. It is an essential concept because it represents the pressure that effectively promotes air flow and distends the lungs. At end-inspiration, if respiratory flow equals zero and there is a clear plateau of the airway pressure, end-inspiratory PL = Pplateau – end-inspiratory Ppleural represents the pressure acting across the alveolar units. At end exhalation, that pressure would be represented, in the absence of auto-PEEP, by end-expiratory PL = PEEP – end-expiratory Ppleural. The importance of the concept of PL becomes clear as the inspiratory PL is the pressure which provides tidal ventilation at the same time that could produce stretch lung injury; and the end-expiratory PL is that required to prevent lung collapse at end-exhalation by being kept positive. Unfortunately, the assessment of PL is not usual in clinical practice because measurement of pleural pressure is not simple, in contrast to measurement of airway pressure. Yet, pleural pressure may be estimated using an esophageal balloon, which is either standalone or attached to an esophagogastric tube and positioned in the lowest third of the esophagus23–25 (Fig. 5.6). These esophagogastric tubes are generally 100 cm long, and the average depth for a correctly positioned balloon is approximately 35 to 45 cm. The catheter is connected to a pressure transducer via a three-way stopcock, and the balloon is inflated to a standard volume depending on the device, which is necessary to prevent artifact because of passive recoil of the balloon walls. Further, the balloon should be long enough to avoid regional variability, providing an average measurement of esophageal pressure to estimate pleural pressure. Although use of an esophageal balloon represents an improvement in the accuracy of pulmonary pressure monitoring, it is not without its limitations. Artifact may arise from mediastinal weight, gravitational gradients in pleural pressure, and airway closure at end exhalation.23 Furthermore, there is considerable controversy in the literature on whether the esophageal pressure measurements should be directly taken as absolute estimates of pleural pressures for PEEP adjustments,26 or whether changes in esophageal pressures should be used to compute chest wall and lung elastances, which are then used to set the level of the administered PEEP.27 Although a ventilator strategy guided by the transpulmonary pressure measured with esophageal balloons improved oxygenation and compliance in a small group of acute respiratory distress syndrome (ARDS) patients,28 a subsequent large trial on patients with moderate to severe ARDS resulted in no significant difference in death and days free from mechanical ventilation between patients receiving an esophageal balloon-guided PEEP and those receiving empirical high PEEP-FiO2 settings.29 Those findings did not support esophageal balloon-guided PEEP titration in ARDS. In critically ill patients with class 3 obesity (body mass index [BMI] ≥40 kg/m2) and ARDS, a retrospective analysis compared a standard protocol of ventilator settings determined by the ARDS net table for lower PEEP/higher FiO2 with a “lung rescue” management strategy with settings determined by an individualized protocol based on lung recruitment maneuvers, esophageal manometry, and hemodynamic monitoring.29 Patients receiving the standard protocol had almost double the 28-day mortality compared with the lung rescue cohort (31% vs. 16%, P = .012; hazard ratio [HR], 0.32; 95% confidence interval [CI] 95%, 0.13–0.78). In robotic-assisted laparoscopic surgery, esophageal manometry can help delineate the portion of increased driving pressure, which is applied to the lungs versus that applied to the chest wall; the increase in elastance associated with pneumoperitoneum is substantially greater for the chest wall (ECW) than for the lungs (ELS).30 This may improve the ability to maintain open lung by appropriately titrating PEEP in this and other perioperative conditions with high susceptibility to lung derecruitment. Driving pressure (ΔP) is the difference between the plateau pressure (Pplat) and PEEP (Fig. 5.7). The driving pressure can also be expressed as the VT normalized by respiratory system compliance (Crs)—that is, the ΔP is proportional to the change in volume of the lung in a breath (VT) divided by its initial volume, to the extent that Crs changes in proportion to lung volume. Change in volume divided by initial volume is, by definition, the volumetric strain. Thus physiologically, the reason why ΔP may be important is because it provides a rough measure of the global volumetric strain of the lungs.31–33 Recent studies suggest that such physiologic considerations may be very important in the clinical setting. Driving pressures have been shown to be better associated with relevant clinical outcomes in ARDS32,34 and surgical patients33,35,36 than VTs. Indeed, a direct association of increased intraoperative ΔP with increased risk of significant postoperative pulmonary complications has been shown in patients undergoing noncardiothoracic surgery, in the absence of a corresponding association with VT33,35, in addition the relative risk of in-hospital death in relation to plateau pressure and driving pressure is depicted in Fig. 5.8. Such a relationship was also found in ARDS patients, with a greater association between increased ΔP and mortality compared with that of VT32 (Fig. 5.9). This finding was reinforced in a secondary analysis of observational data on patients with moderate to severe ARDS ventilated with standard lung-protective ventilation.37 Variation in VT or PEEP had no impact on mortality. In contrast, above a plateau pressure of 29 cm H2O or a driving pressure of 19 cm H2O, there was an ordinal increment in risk of death.37 In thoracic anesthesia, comparison of conventional lung-protective ventilation (one-lung ventilation [OLV] with VT = 6 mL/kg ideal body weight, PEEP 5 cm H2O, and recruitment maneuvers) with ΔP-guided ventilation (same VTs and recruitment, individualized PEEP to produce the lowest ΔP during OLV) showed a smaller incidence of pneumonia or ARDS in the ΔP-guided group.38 A retrospective analysis of data of patients undergoing OLV for thoracic surgery (hospital electronic medical records and the Society of Thoracic Surgeons database) indicated that ΔP, when modeled together with VT, predicted major morbidity, whereas VT alone inversely correlated with respiratory complications.39 This implied that low VT by itself was not associated with less postoperative pulmonary complications, whereas low VT taken together with strategies to minimize ΔP may be.39 The lungs and the chest wall have elastic properties that tend to restore them to their combined equilibrium configuration when they are displaced from baseline by spontaneous or mechanical ventilation. Thus if the pleural space is open at FRC, the lungs would recoil inward toward collapse, while the chest wall would recoil outward, toward expansion. Elastance is the change in distending pressure for a given change in volume (E = ΔP/ΔV). The elastance of the total respiratory system (ERS) is the sum of the lung (EL) and the chest wall (ECW) elastances: Compliance is the reciprocal of the elastance (C = ΔV/ ΔP); that is, the change in volume for a given change in distending pressure. Compliance tends to be more frequently used clinically. Thus the earlier equation can be alternatively expressed as: where CRS, CL, and CCW are the compliances of the respiratory system, lung, and chest wall, respectively. During mechanical ventilation with volume control, a peak inspiratory pressure (PIP) is usually seen, which is followed by a plateau pressure (Pplat) if an inspiratory pause (i.e., a period of no flow at end of inspiration) is provided (see Fig. 5.7). The difference between peak and plateau pressures is caused by dynamic factors, particularly airway resistance. Dynamic compliance (Cdyn) is defined as the compliance calculated using the PIP: where PEEP is the positive end-expiratory pressure (see Fig. 5.7). Cdyn, thus, conflates the distensibility of the lungs themselves with the resistance of the airways, in addition to the properties of the chest wall, to the overall quantification of compliance. Static compliance (Cstat) aims to eliminate the dynamic airflow-dependent component from the computation of compliance by using Pplat: Lung compliance represents the distensibility of the lung parenchyma; it is altered in various disease states. Compliance is decreased with consolidation, atelectasis, pulmonary edema, interstitial disease or fibrosis, pneumothorax, surgical resection, large effusion, and mainstem intubation. It is increased in emphysema because of loss of elastic tissue. In addition, lung compliance depends upon the lung volume in a nonlinear manner. The highest lung compliance is observed when lungs are at moderate volumes, and lowest at the extremes of low or high lung volumes. Such relationships are expressed in the pressure-volume curve of the lung (Fig. 5.10). That curve also shows that the pressure-volume relationship and the compliance for the same volume (or pressure) on inhalation differs from that on exhalation, a phenomenon known as hysteresis. To compute the compliance of the lungs, the contribution of the chest wall needs to be subtracted. Accordingly, lung compliance (CL = ΔVLΔPL) can be estimated as: ,where PawEI is the airway pressure at end inhalation, PesEI is the esophageal pressure at end inhalation, PawEE is the airway pressure at end exhalation, and PesEE is the esophageal pressure at end exhalation, measured using esophageal manometry, as explained earlier. Chest wall compliance reflects the distensibility of the muscles, bones, and soft tissue that comprise the chest wall. Chest wall compliance is reduced with increased abdominal pressure (e.g., insufflation, abdominal compartment syndrome), chest wall edema, thoracic deformity, muscle tone, extensive thoracic/abdominal scar, supine positioning, and obesity. Chest wall compliance is increased with flail chest, muscle relaxation, and upright positioning. Using estimates of pleural pressure, the compliance of the chest wall (CCW) can be calculated as: with variables that have been defined earlier. Resistance is the opposition to fluid flow because of friction and may be calculated as the ratio of the driving pressure to air flow (Raw = ΔP/ where ρ is the fluid/gas density (kg/m3), u is the velocity (m/s), L is the length (m), and μ is the viscosity (kg/ms). Laminar flow dominates at Re less than 2300, while turbulent flow dominates at Re greater than 2900. Laminar flow through a long cylindric pipe is governed by Poiseuille’s Law, which is appropriately applied to conducting airways. Resistance is relatively low in laminar flow, and is calculated as follows: where L is the length, u is the velocity, and r is the radius. Thus the airway radius is especially important in determining the resistance; if the radius is halved, the resistance increases by a factor of 16. Pathologic states, such as tracheal stenosis and bronchospasm, raise the resistance and increase the work of breathing. Reducing the density of the gas mixture (e.g., through mixing with helium, “Heliox”) can improve resistance and lead to decreased work of breathing. Because of the dependence on radius, an individual small airway has a higher resistance than a large airway. However, because the airways branch so prolifically, the overall resistance depends on the number of parallel pathways present, and it is the medium-sized airways that have the overall greatest resistance (see Fig. 5.2). Stress is defined as force divided by the area over which it is applied.31 Lung stress can be estimated through the transpulmonary pressure (PL). This will be larger at end-inspiration and lower at end-exhalation, in the absence of patient effort. Strain is the change in dimension relative to the material’s initial dimension. Based on this definition, lung volumetric strain can be calculated as the ratio of the change in lung volume (dV) to the resting lung volume (V0), that is, dV/V0.40 Assuming a linear relationship, lung stress and strain are related to one another by the specific lung elastance, K. . Experimental studies indicate that when stress and strain are outside the normal physiologic limits, the lung releases inflammatory cytokines and mediators, which indicate, produce, and exacerbate lung injury. Very large VTs conducive to large strains, either through spontaneous or mechanical ventilation, can produce lung injury.41,42 When mechanical ventilation is applied for more than 48 hours in initially normal animal lungs, injury occurs when lung expansion reaches approximately the TLC, that is when strain would be (TLC – FRC)/FRC.40,43,44 In experiments representing usual clinical conditions, those large values are not present.45 This suggests an increased susceptibility of lung tissue to strain in the presence of an additional injurious trigger (i.e., systemic inflammation).46,47 A large animal study modeled moderate–severe ARDS (respiratory system compliance = 14.0 ± 4.9 mL/cm H2O; PaO2/FiO2 = 92 ± 59) with surfactant depletion and ventilator-induced lung injury (VILI) and quantified volumetric strain using CT to support a relationship between strain and local inflammation assessed with positron emission tomography (PET) in those extreme lung injury cases.48 Stress is approximated by the transpulmonary pressure distending the airspaces, but the regional stress of the lung will vary because of gravity and other factors producing heterogeneous lung expansion. This will produce a distribution of stress throughout the lungs. For example, in uniformly expanded lungs, alveolar pressure is the same across the whole lung, but when the lung is not uniformly expanded (such as when air spaces are isolated from one another because of obstruction or closure), alveolar pressures will not be uniform throughout.49 The presence of such heterogeneity leads to regional stress values substantially larger than those present at the global level.45 This means that even when global transpulmonary pressures are low, local stresses can be high, even to the limit of being injurious.45,50
Pulmonary Pathophysiology and Lung Mechanics in Anesthesiology
Abstract
Keywords
Introduction
Essentials of Lung Structure
Tracheobronchial Structure
Respiratory Airways
Lung Volumes
Nomenclature
Tidal Volume
Residual Volume
Expiratory and Inspiratory Reserve Volumes
Functional Residual Capacity
Total Lung Capacity
Closing Capacity and Closing Volume
Effects of Anesthesia
Respiratory Mechanics
Transpulmonary Pressure
Driving Pressure
Elastance and Compliance
Resistance
). Airway resistance is determined by the dimensions of the airway, the viscosity of the gas, and whether the flow is laminar or turbulent, which in turn is predicted by the Reynold’s number, Re:
Stress and Strain
Full access? Get Clinical Tree
Pulmonary Pathophysiology and Lung Mechanics in Anesthesiology
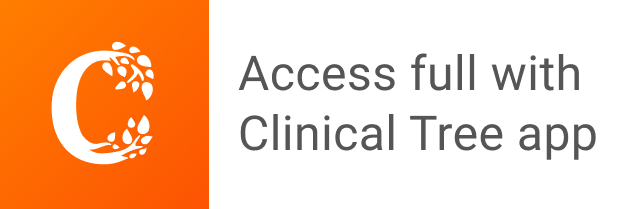