Chapter Outline
History, Philosophy, And General Comments 41
Clinical Practice Using The Closed Circuit Technique 42
Vapor Delivery Techniques 42
Free Water Requirements 43
Physiological Aspects Of Closed Circuit Anesthesia 43
Pediatric Considerations 44
Accumulation of Gases 44
Physiological Monitoring Considerations 45
Appendix A: Quantitative Calculations 56
History, Philosophy, and General Comments
Very low flow and closed circuit anesthetic techniques are predicated upon a practical method for the absorption of carbon dioxide and therefore the prevention of CO 2 rebreathing. Between 1935 and 1950, most anesthesiologists were quite familiar with closed circuit anesthetics using cyclopropane and Waters’ granular 4 to 8 mesh pellets. Low flow and closed circuit anesthesia eliminate the spillover of oxygen and explosive gases into an operating room with electrocautery and static electricity.
The practice of closed circuit anesthesia became less common following the introduction of halothane in the 1950s and was replaced by the semiclosed circle absorption technique using a variety of fresh gas flows (FGF), often 5 L/min or greater. Yet the basic principles are easy in concept and implementation: the closed circuit technique emphasizes the maintenance of a constant anesthetic state by the addition of gases and vapors to the breathing circuit at the same rate that the patient’s body redistributes, stores, and eliminates them. While the open or semiclosed anesthetic relies on progressively diminishing delivered concentrations to maintain a desired alveolar and arterial concentration of anesthetic, the closed circuit anesthetic approach emphasizes the amount of vapor or gas needed to maintain that constant alveolar and arterial concentration.
The successful use of potent inhalation agents in a closed circuit requires the repeated administration of a calculated unit dose at sequential time intervals that increase by the square of time. The clinical skills needed are identical to those required for any anesthetic practice—vigilance, diligence, and attention to detail. Moreover, a sound fund of knowledge about physiology, pharmacology, and equipment remains the basis for the close adjustments required for the care of each individual patient. The calculations are simple: when the patient plus the anesthesia machine are viewed as interdependent, a combined “prime” for the breathing circuit and the patient followed by a unit dose for the patient can be delivered at predictably lengthening time intervals to attenuate the surgical stress response and keep the patient at basal levels of oxygen consumption ( Appendix A ).
The advantages of this concept include:
- 1.
The patient constantly providing information about his metabolic state using the anesthesia machine as a monitor of metabolic oxygen consumption ( <SPAN role=presentation tabIndex=0 id=MathJax-Element-1-Frame class=MathJax style="POSITION: relative" data-mathml='V·’>V·V·
V ·
o 2 ). The quantitative nature of the practice also evaluates carbon dioxide production (V co 2 ), cardiac output, and free water requirements.
- 2.
A relative humidity of 40% to 60% without additional connections or the use of valveless breathing systems used at partial rebreathing flows. Thus, mucociliary function may be better preserved.
- 3.
Heat is conserved from the patient and supplied because of the exothermic reaction of the carbon dioxide absorbent.
- 4.
Adequacy of the circulation can be assessed by monitoring <SPAN role=presentation tabIndex=0 id=MathJax-Element-2-Frame class=MathJax style="POSITION: relative" data-mathml='V·’>V·V·
V ·
o 2 .
- 5.
Adequacy of neuromuscular blockade can be assessed by monitoring the ventilator bellows or the rebreathing bag.
- 6.
Economy—decreasing the total milliliters of anesthetic agent administered using low flow techniques can save hundreds of millions of dollars annually in the United States, and upwards of half a billion dollars worldwide.
- 7.
Environmental responsibility—with a lower total amount of chlorinated hydrocarbon anesthetics used, the contribution we make to ozone layer depletion will be less. Chlorine-free anesthetics such as desflurane can be used less expensively.
- 8.
The use of nitrous oxide for the administration of anesthesia or analgesia accounts for 3% to 12% of global nitrous oxide release. With a lower total amount of nitrous oxide used, ozone layer depletion will be decreased.
Only with flows less than 2.0 L/min does the rebreathing fraction reach 50% or more. It is useful, therefore, to start with some definitions:
Low flow technique : less than 1.0 L/min (where the rebreathing fraction is >50%)
Minimal flow technique : 0.5 L/min
Closed circuit technique : a fresh gas flow not to exceed the gas loss via patient uptake plus system leaks. May be nonquantitative (with the administration of volatile anesthetic agent according to clinical assessment) or quantitative (with the administration of volatile anesthetic agent according to calculated values based on prime dose plus unit dose administered according to the square root of time model).
Clinical Practice Using the Closed Circuit Technique
Proper equipment for a closed circuit technique includes an anesthesia machine and the appropriate circuit delivery components, which typically are a circle system passing through a carbon dioxide absorber.
CO 2 Absorption
Understanding the chemistry of CO 2 absorption is the basis of confidence in the closed circuit technique. CO 2 absorption is a chemical reaction of a base neutralizing an acid. The acid is carbonic acid formed by the reaction of CO 2 and water; the base is the hydroxide of an alkali or alkaline earth metal. The end products of the exothermic reaction are water, heat, and a carbonate. The most common absorbent is soda lime; Baralyme (barium hydroxide) is no longer available. A 1.13 kg canister of soda lime should last approximately 15 hours with 0% breakthrough or 16.3 hours with only 0.5% CO 2 breakthrough.
Machine Construction
The ideal machine will have a leak-free gas delivery system, breathing circuit, and ventilator. Low gas flow capability (i.e., low-flow calibrated flowmeters) is highly desirable, and a mechanical ventilator tidal volume that is not related to the circuit volume. A tight system (i.e., leak less than 100 mL/min at 30 cm H 2 O pressure) is required.
Anesthetic Uptake
The uptake of volatile agents is rapid in the first few minutes of the anesthetic and therefore attaining a clinically satisfactory alveolar concentration may be more problematic. The less soluble the agent, such as the newer agent desflurane (introduced in 1992) and sevoflurane (introduced in 1995), the less this is a problem. Although experience has been gained worldwide with closed circuit administration of sevoflurane, current FDA regulations and the package insert state “to minimize exposure to compound A, sevoflurane exposure should not exceed 2 minimal alveolar concentration (MAC) hours at flow rates of 1 to less than 2 L/min. Fresh gas flow rates less than 1 L/min are not recommended,” so we will restrict this discussion to desflurane.
The anesthetic potency of desflurane is low, with an MAC between 4% and 8%, depending on the age of the patient; a comparatively high alveolar concentration is therefore required for maintenance. Desflurane is metabolized at a virtually negligible rate of approximately 0.02%. The wash-in of desflurane is so rapid that within 10 minutes after induction the inspired desflurane concentration is about 85% of the fresh gas concentration. Even with extremely low fresh gas flows, the difference between inspired and fresh gas concentration is comparatively small and the desflurane concentration can be readily increased.
While one may get started with an intravenous or mask induction, during the first 10 to 20 minutes, a semiclosed circuit fresh gas flow of several liters should be used, and then reduce the flow thereafter. A closed circuit requires a reasonable atmospheric seal and most patients would prefer not to have a tight-fitting mask while conscious. Moreover, a volatile agent induction at closed circuit flows is impractical because the fresh gas flow rate will not be sufficient to vaporize an induction dose.
Vapor Delivery Techniques
It doesn’t matter whether the agent is vaporized in a vaporizer or in the circuit itself by liquid injection; similar mean circuit concentrations will be produced according to the technology of the vaporizer and the settings determined by the anesthetist or to the gas laws at standard temperature and pressure (STP).
Vaporizer Techniques
Once the patient is induced and the trachea intubated, high flow rates (e.g., FGF of 4 to 6 L/min) should be used for the first 10 to 15 minutes to (1) flush and prime the circuit and functional residual capacity (FRC) and (2) to provide for early uptake in the rapid saturation phase. The FGF is then decreased progressively over the first half hour (in adults) to approximately 250 mL/min O 2 and 250 mL/min N 2 O (unless N 2 O is not used as an agent). The N 2 O can then be decreased according to the adequacy of circuit volume, best detected by the rise of ventilator bellows to “almost fill” or the rebreathing bag to “almost full.” The setting on the vaporizer typically used at this time is that setting required to maintain an adequate end-tidal anesthetic level, typically 1.3 MAC. It would not be unusual, for example, to have the vaporizer set to 4% and read an end-tidal level of 1.5%. Fifteen to 30 minutes before the end of the surgery, the N 2 O (if used) and agent may be shut off with only the oxygen flowing at the closed circuit rate; it is usually possible to “coast” in this fashion for the last 15 to 30 minutes of the case. If a rapid change in circuit vapor concentration is required, flows may be transiently increased. Care should be taken, however, that if the vaporizer is already set high, when flows are increased, much higher blood vapor concentrations will result in a short time.
Injection Techniques
The liquid anesthetic agent can be introduced into either limb of the breathing circuit and will vaporize in a fashion predicted by the gas laws. Usually the expiratory limb is chosen because of the greater distance and volume of gas between the injection port and the patient. This affords an additional buffer of circuit volume at the expense of a longer time interval to the desired alveolar concentration of anesthetic agent.
Free Water Requirements
Water is required for the elimination of heat generated during metabolic activity, and water loss is normally 100 mol for every 100 kcal expended. Free water requirements are ultimately proportional to caloric demands, which are decreased when patients are anesthetized. Basal water requirements can therefore be calculated in relation to basal metabolic demand:
- 1.
The consumption of 1000 mL of oxygen generates 4825 calories.
- 2.
If <SPAN role=presentation tabIndex=0 id=MathJax-Element-3-Frame class=MathJax style="POSITION: relative" data-mathml='V·’>V·V·
V ·
o 2 is equal to 10 × kg 3/4 (mL/min) in adults, then heat production per hour is:
- 3.
<SPAN role=presentation tabIndex=0 id=MathJax-Element-4-Frame class=MathJax style="POSITION: relative" data-mathml='10×kg3/4×4825×601000′>10×kg3/4×4825×60100010×kg3/4×4825×601000
10 × kg 3 / 4 × 4825 × 60 1000
- 4.
The calories required for 1 mL of water to evaporate are 63 cal. This plus 540 calories are required to achieve the heat of vaporization (total 603 cal/mL of water). This modifies the above equation to: mL water/hour = <SPAN role=presentation tabIndex=0 id=MathJax-Element-5-Frame class=MathJax style="POSITION: relative" data-mathml='10×kg3/4×4,825×601000×603≈5×kg3/4′>10×kg3/4×4,825×601000×603≈5×kg3/410×kg3/4×4,825×601000×603≈5×kg3/4
10 × kg 3 / 4 × 4,825 × 60 1000 × 603 ≈ 5 × kg 3 / 4
which parallels basal oxygen consumption once again.
Physiological Aspects of Closed Circuit Anesthesia
Monitoring <SPAN role=presentation tabIndex=0 id=MathJax-Element-6-Frame class=MathJax style="POSITION: relative" data-mathml='V·’>V·V·
V ·
o 2 , V co 2 , Cardiac Output, and Respiratory Mechanics
With the volume of the system constant and the oxygen concentration maintained in a steady state as monitored by an oxygen analyzer, the oxygen flow required becomes a monitor of oxygen consumption. Basal oxygen consumption ( <SPAN role=presentation tabIndex=0 id=MathJax-Element-7-Frame class=MathJax style="POSITION: relative" data-mathml='V·’>V·V·
V ·
o 2 ), CO 2 production (V co 2 ), free water requirements, and the cardiac output can be estimated by the “ 3/4 power law” (Brody’s number).
For example, if Brody’s number was calculated for a 70 kg adult:
Body weight (kg) | 70.0 | |
Brody’s No. | kg 3/4 | 24.2 |
Oxygen consumption ( <SPAN role=presentation tabIndex=0 id=MathJax-Element-8-Frame class=MathJax style="POSITION: relative" data-mathml='V·’>V·V· V · o 2 ) (mL/min) | Brody’s No. × 10 | 242.0 |
CO 2 production (V co 2 ) (mL/min) | Brody’s No. × 8 | 193.6 |
Free water requirement (mL/hr) | Brody’s No. × 5 | 121.0 |
Cardiac output (dL/min) | Brody’s No. × 2 | 48.4 |
The minimum flow rate of 250 mL/minute established for anesthesia machines, when calculated for a 70 kg adult, is equal to 10.5 × kg 3/4 . Oxygen consumption calculated from Brody’s number might underestimate the true oxygen consumption at the start of the case due to patient anxiety and the release of endogenous catecholamines. Given a steady-state relationship established between the anesthesia machine and the patient, an increase in mean circuit oxygen concentration reflects an excess of O 2 supply compared with metabolic demand. Likewise, a decrease in mean circuit oxygen concentration reflects an increase in the patient’s oxygen demand.
The rate of CO 2 production will be related to the rate of oxygen consumption by the respiratory quotient (RQ = 0.8 normally) and can aid in the calculation of minute ventilation requirements.
For example, if we calculate the minute volume required for normocarbia:
Brody’s No. | kg 3/4 | 24.2 |
Minute CO 2 production (mL/min) | Brody’s No. × 8 | 193.6 |
Normocarbia (% atm) | 5% | |
V A for normocarbia (mL/min) | V co 2 /.05 | 3872.0 |
Ventilatory requirements significantly out of proportion to “normal” or expected amounts may accompany a hypometabolic or hypermetabolic state, such as hypothyroidism or malignant hyperthermia.
Human studies have verified subgroups of patients compensating for an oxygen debt, for example, following the release of an aortic cross clamp after aortic aneurysmorrhaphy. This was monitored by a decrease in mean circuit O 2 concentration accompanied by an increase in cardiac output. Intriguingly, the linear correlation between body mass and oxygen consumption established with painstaking detail in animal models by Brody is the basis of the concept of oxygen debt in experimental models and the early studies of survival of critical illness in humans.
For anesthesia machines with a ventilator bellows, the bellows themselves become a sensitive monitor of diaphragm activity when filling just before the next ventilatory cycle, as respiratory efforts can easily be detected by watching bellows movement. Moreover, the bellows of a low-flow system more accurately reflects the expiratory flow rate because the patient’s exhalation volume provides the bulk of the gas in the bellows—the rate of ascent of the bellows directly reflects the exhalation flow rate of the patient. Bronchospasm or impaired exhalation is more easily detected and the fill rate of the bellows more easily monitors the success of therapeutic interventions. Finally, movement of the bellows is a sensitive monitor of the degree of neuromuscular blockade because the diaphragm recovers from neuromuscular blockade before the peripheral muscles.
Defense Against Humidity and Temperature Loss
Gases entering the breathing system (and therefore the patient) are anhydrous. In contrast, at the normal body temperature of 37 ° C, the saturated vapor pressure is 47 mm Hg. Thus humidification of respiratory gases has been recommended for the prevention of pulmonary damage, maintenance of normal ciliary motility, and for the maintenance of body temperature. Humidification well within the recommended range of 14 to 30 mg H 2 O/L is readily achievable in a short time using a closed circuit technique and compares favorably with other partial rebreathing ventilation methods or the use of heat and moisture exchangers ( Figure 4–1 ).

Similarly, advantage can be taken of the exothermic reaction of CO 2 absorption by soda lime during a closed circuit anesthetic, as heat is liberated at the rate of 13,700 calories per mole of water produced. In addition, a lower fresh gas flow rate will allow the patient to not lose heat to the incoming bulk of cold, dry fresh gas.
Pediatric Considerations
Energy requirements of children are greater than those of adults, even more than would be predicted by the exponential nature of Brody’s equation. Sarrus and Rameaux proposed that metabolic values in homeotherms were likely an exponential function more than 150 years ago. They went on to suggest that not only was oxygen consumption related to heat production and surface area, but it was also related to respiratory rate, heart rate, pulse volume, and body size. Among many authors who extended this proposition, Kleiber reasoned that if heat loss is proportional to surface area, then heat production must also be proportional to surface area, since homeothermia dictates that heat production equals heat loss.
To complicate matters a little further, in children, RQ may be influenced by the anesthetic. For babies weighing less than 10 kg, a reduced V co 2 in relation to <SPAN role=presentation tabIndex=0 id=MathJax-Element-9-Frame class=MathJax style="POSITION: relative" data-mathml='V·’>V·V·
V ·
o 2 has been noted, yielding respiratory quotients that sometimes were lower than 0.7. This may result from partial inhibition of brown adipose tissue function caused by halothane. In an in vitro model, halothane, isoflurane, and enflurane were approximately equipotent inhibitors of thermogenesis, with concentrations of approximately 0.7% resulting in 50% inhibition.
Additional challenges may occur when using a closed circuit technique during pediatric anesthesia.
- ▪
The use of cuffless endotracheal tubes with a variable leak from 15 to 30 cm H 2 O will result in volume loss to the atmosphere and decreased volume return to the breathing circuit. Throat packs may be used to seal the leak without using a larger tube or a cuffed tube. On the other hand, small-sized cuffed endotracheal tubes are perfectly safe when attention is given to not exceeding an intracuff pressure of approximately 25 mm Hg, or a pressure just sufficient to prevent an air leak.
- ▪
Flowmeters , even when calibrated to 50 mL/min FGF, may be insufficiently precise for delivering very low fresh gas flow rates.
- ▪
Relatively small arterial doses in comparison to the breathing circuit and ventilator prime doses compel practitioners to precisely calculate pediatric dosing requirements for the closed circuit lest an overdose occur. Couto da Silva found that pediatric patients typically required higher volume and more frequent dosing of volatile agents than predicted on theoretical grounds, which parallels Lindahl’s recommendation of increasing the constant “k” multiplier of Brody’s number by 40% for children.
Potential Problems
Because gases that would ordinarily be flushed out continuously during the administration of higher FGF anesthetics remain within the breathing circuit, accumulation of toxic substances is of potential concern during closed circuit practice.
Accumulation of Gases
Metabolic by-products of halothane have been detected in very low concentrations in the closed circuit; however, they do not appear to be of clinical significance. Ethrane isoflurane , and desflurane have not been found to have significant metabolic by-products. Sevoflurane produces a variety of degradation products with the use of soda lime and baralyme. In the short term (cases up to 12 MAC hours in duration), compound A accumulation does not appear to be a clinical problem. Mean compound A concentrations in the inspiratory and expiratory limbs of the circle are significantly different at FGF rates of 0.5 and 2.0 L/min. The quadrupling of the FGF rate was not associated with a significant difference in compound A volume within the circle in one study, while in general there appears to be some FGF dependence of compound A circuit concentrations.
The FRC (of an adult) contains about 2 L of nitrogen, and the breathing circuit contains about 4 L of nitrogen. In addition, tissue nitrogen is released into the breathing circuit as progressive denitrogenation occurs. The amount of nitrogen dissolved in tissues of the average adult is about 1 to 1.5 L. Even if all this nitrogen were to pass into the functional residual capacity and recreating system, its inspired concentration would increase to, at most, 10% to 15%.
Acetone, methane , and other gases have also been detected in the breathing circuit, prompting the suggestion that the closed circuit should be flushed briefly but periodically with higher FGFs. Methane, up to 100 parts per million (ppm), is excreted in expired gas. Intestinal organisms produce it. Blood methane levels of 2000 ppm (0.02%) have been recorded without apparent clinically significant effect. Acetone is produced by hepatic metabolism and may accumulate in patients with preoperative starvation or increased production (diabetes, cirrhosis). Concentrations greater than 50 ppm may cause nausea, vomiting, and slow emergence from anesthesia.
Carbon monoxide production occurs endogenously during the degradation of red cell hemoglobin to bile pigments when a carbon atom is separated from the porphyrin nucleus and is subsequently catabolized by heme oxygenase. Any disturbance leading to accelerated destruction of RBCs and accelerated breakdown of other hemoproteins would lead to increased production of CO. Hematomas, intravascular hemolysis of RBCs, blood transfusion, and ineffective erythropoiesis all will elevate carboxyhemoglobin (COHb) concentration in blood. In females, COHb levels fluctuate with the menstrual cycle; the mean rate of CO production in the premenstrual, progesterone phase is almost doubled. Neonates and pregnant women also show a significant increase in endogenous CO production related to increased breakdown of RBCs. COHb formation as a hemoglobin breakdown product occurs with a normal physiological range of 0.4% to 0.8% (somewhat higher findings in urban dwellers).
There is a constant rise of carboxyhemoglobin during a closed circuit anesthetic, approximately 0.05 g/100 mL of blood over 6 hours in adults. The rate of rise is equal in smokers and nonsmokers, although their baselines are different. During ventilation with high flows, carboxyhemoglobin levels decrease. Patients for whom this may be a potential problem are those with anemia, severe coronary artery disease or peripheral vascular disease, or increased levels of endogenous carbon monoxide production such as pregnant women, newborns, those with hemolytic disease, and porphyria cutanea. Prolonged exposure of desflurane, enflurane, and isoflurane to desiccated CO 2 absorbents may result in anesthetic degradation, leading to the production of CO. The use of dry absorbent produces more CO than standard absorbent with normal amounts of water. Increased temperature increases CO production, as does the use of higher anesthetic concentrations. To minimize exposure to CO from the degradation of anesthetics in the breathing system, only an absorbent with the full complement of water should be used.
Physiological Monitoring Considerations
Carbon Monoxide Toxicity Monitoring
Pulse oximetry is inadequate as a safety monitor with regard to COHb monitoring, since the commonly employed sensors do not differentiate between O 2 Hb and COHb with the two wavelengths of light used. Under anesthesia, alterations in mental status or the development of respiratory distress cannot be detected. Skin color is not accurate since COHb has a red color and mild acidosis increases skin perfusion. Thus unexplained ECG signs of myocardial hypoxia (ST segment changes, T wave inversion, rhythm disturbances) or an unexpected acidosis or base deficit despite normovolemia should prompt further diagnosis. CO saturation can be measured with blood gas analyzers employing light of at least five different wavelengths. The detection of CO in the circle system can be performed by electrochemical devices used in toxicological studies; however, there are numerous technical challenges in relating ambient air CO levels to blood CO levels, let alone tissue levels such as brain or myocardial CO.
Sidestream Bulk Gas Monitoring
Sidestream respiratory gas monitoring potentially complicates the use of closed circuit techniques because of sample gas withdrawal and therefore circuit volume loss at rates from 50 to 200 mL/minute, depending on the specifications of the particular gas analyzer. Mainstream infrared analysis without bulk gas withdrawal is one solution. An alternative is the return of sampled gas from the exhaust valve of the capnograph back to the breathing circuit. Adding fresh gas at identical gas composition to offset the loss of circuit gas is a third option. This is of questionable clinical significance in adult patients, but may become important for children.
Emergence
As a corollary to the “quantitative” uptake and distribution concepts of closed circuit practice, the rate of decline of residual agent will be related to the duration of the anesthesia itself (i.e., after 121 minutes of anesthesia (11 2 ), another unit dose will result in approximately 23 minutes of anesthesia (12 2 − 11 2 = 144 − 121). To the extent that one does not inject during that time, one can “coast” to the next square of time interval, with an expected decrease in end-tidal agent concentration. FGF will be another means of affecting the end-tidal agent concentration, in that a higher FGF will “flush” residual agent in the ventilatory portion of the system. If the patient is waking up too fast, the FGF can be reduced and the agent will equilibrate from tissue stores. The usual clinical signs of emergence should be followed such as patient movement, pupil size, vital signs, respiratory tidal volume (if breathing spontaneously), abdominal tone (if muscle relaxants are not used), and end-tidal agent levels. The Bispectral Index (BIS) or the Entropy monitor may also be helpful.
A charcoal shunt on the inspiratory limb may be used to facilitate emergence while maintaining a closed circuit. A reservoir containing 50 g of activated charcoal (carbon processed to make it extremely porous and thus have a very large surface area available for adsorption) can be incorporated into the inspiratory limb of the circuit to adsorb volatile anesthetic agents (without adsorbing oxygen or nitrous oxide). Inspiratory levels of volatile agents are reduced with incorporation of the charcoal shunt.
Closed Circuit Anesthetic Record Keeping
Recording the output setting of the vaporizer at closed circuit flows is meaningless other than to satisfy the curiosity of the anesthetist about the unreliability of vaporizer output and low FGF.
At FGFs below 1 L/min, one should record inspired and expired agent concentrations on separate lines of the anesthesia record when an agent-specific vaporizer is used. Alternatively, with injection methods, one may simply wish to record only the end-tidal agent level.
Cost Containment Considerations
Closed circuit anesthesia is one of the easiest ways to reduce anesthesia costs. The costs of inhalation anesthesia are primarily related to the agent selected, the concentration delivered, the duration of the anesthetic, and the total FGF rate. As a rough guideline for adult practice, the agent used in milliliters per hour is approximately the FGF in liters per minute times three times the percent of the agent. Therefore an FGF of 5 L/min with 2% isoflurane uses approximately 30 mL/hr of liquid isoflurane; an FGF of 1 L/min and 2% isoflurane uses approximately 6 mL/hr of liquid isoflurane.
Appendix A
Quantitative Calculations for Dosing the Closed Circuit by Injection Techniques
Dosing Frequency
In 1954, Severinghaus suggested that the rate of rise and uptake of anesthetic gases and oxygen was predictable according to a square root of a time-based model, visually expressed as the familiar tissue uptake curve. These calculations became the basis for the “square root of time” model for quantitative dosing of the closed circuit:
Administration rate = Uptake = kt -1/2
Lowe confirmed this mathematical model pharmacologically with halothane arterial blood concentration analysis and also pointed out that the time between injections is the sequence of odd integers (1, 3, 5, 7… minutes). This is best illustrated by the overlaying of a typical vapor delivery curve on the above tissue distribution curve, which in either event should achieve a constant alveolar concentration ( Figure 4–2 ).

Identical areas under the curve at square root of time progressively lengthening intervals are responsible for the clinical “steady state,” when the gas machine, breathing circuit, patient’s lungs, heart, and end organs are viewed as a unitary delivery and uptake “system.” The amount of anesthetic vapor required to fill this system is the sum of what is required to fill the ventilatory portion and arterial transport system at time “0” (the prime dose ) and the amount required to be added as the anesthetic is absorbed, distributed, and biotransformed through body tissues until time t (the unit dose ). This is the amount that is constant at each additional “square root of time” unit ( Figure 4–3 ).

Knowledge of the physical properties of the inhalation agents is important for calculating the unit dose ( Table 4–1 ). Sevoflurane is excluded because of current Food and Drug Administration regulations regarding its use at closed circuit flows; current recommendations are not to use it at less than 1 L of total fresh gas flow for up to 2 MAC hours.
Agent | mL vapor/mL liquid at 37° C | MAC at 37° C | Λ B/G at 37° C | Vapor Pressure at 20° C |
---|---|---|---|---|
Halothane | 240 | 0.75 | 2.4 | 242 |
Enflurane | 210 | 1.7 | 1.9 | 180 |
Isoflurane | 206 | 1.3 | 1.5 | 250 |
Desflurane | 195 | 4.6 | 0.42 | 735 |
Dose Requirements
Because any alveolar anesthetic concentration (C A ) can be considered a fraction or a multiple ( f ) of MAC, the following equation applies:
C A = f MAC ( in mL of vapor / dL )
Equilibrium is expected to occur in normal alveoli, therefore, the systemic arterial and blood anesthetic concentration (C a ) can be calculated from the alveolar concentration (C A ) provided pulmonary shunts are excluded because λB/G values equate vapor concentrations between gas and blood phases:
C a = C A × λ B / = f MAC × λ B / G ( in mL of vapor / dL )
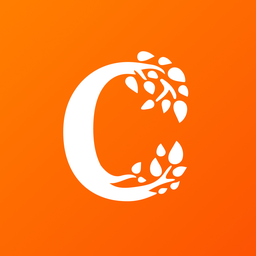
Full access? Get Clinical Tree
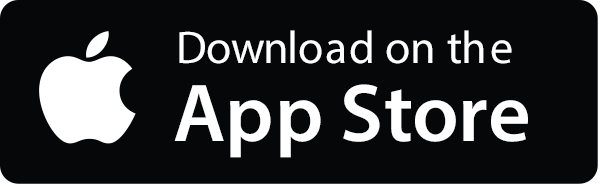
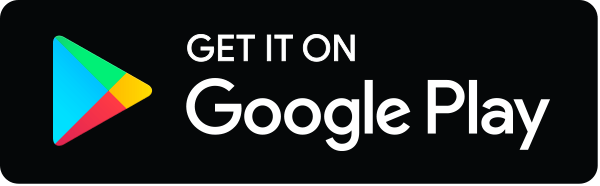