Practical Problems and Complications of Mechanical Ventilation
KEY POINTS
1 Impaired cardiac output is most likely to result from mechanical ventilation when intravascular volume is depleted, vascular reflexes are impaired, and the patient is inflated passively with high mean airway pressures.
2 All forms of alveolar rupture induced by mechanical ventilation—interstitial emphysema, pneumomediastinum, pneumoperitoneum, subcutaneous emphysema, cyst formation, pneumothorax, and systemic gas embolism—have been described in both infants and adults. Certain high- pressure ventilatory patterns are strongly suspected to inflict nonrupture damage, such as diffuse lung injury and bronchopulmonary damage (ventilator induced lung injury).
3 The pathophysiology of tension pneumothorax involves cardiovascular as well as ventilatory compromise. Tension physiology can develop with only a minor portion of the lung collapsed if the lung is infiltrated, the airways are obstructed, or the lung adheres to the chest wall to cause loculations.
4 No single cause is responsible for all barotrauma. High peak airway cycling pressure, necrotizing pneumonia, heterogeneity of lung pathology, copious airway secretions, and duration of positive-pressure ventilation are major predisposing factors. High levels of minute ventilation and cardiac output and very rapid inspiratory flow rates are likely contributors.
5 The thoracostomy tube selected should be of such size and location to adequately drain the pleural air and/or liquid. The lung must be approximated to the parietal pleura whenever possible. Suction may be needed if an air leak is large, if it persists on water seal alone, or if fluid drainage is copious.
6 Failure to limit end-inspiratory plateau pressure and to preserve a crucial minimum level of positive end-expiratory pressure in the early phase of acute respiratory distress syndrome may intensify preexisting alveolar damage, especially when high peak inflation pressures are used. High shearing forces of each tidal cycle may cause small airway damage, capillary stress fracture, and inflammatory changes, as well as overt lung disruption. Later in the disease process, when inflammation has degraded the collagen infrastructure of the lung, minimizing peak pressure helps prevent alveolar rupture, cyst formation, and pneumothorax.
7 Subacute and chronic complications of mechanical ventilation include fluid retention, redistribution of body water, infection, altered ventilatory drive, and respiratory muscle deconditioning.
8 Specific problems encountered after intubation of the critically ill patient include the following: uncontrolled coughing, patient-ventilator asynchrony, excessive work of breathing, and self-extubation. Agitation during mechanical ventilation demands a systematic search through possible causes relating to the patient, the endotracheal tube and circuitry, and the ventilator itself.
▪ ACUTE COMPLICATIONS OF MECHANICAL VENTILATION
Cardiovascular Impairment
Patients with an uncompromised heart, normal sympathetic reflexes, and normal or increased intravascular volume tolerate mechanical ventilation well, especially if spontaneous efforts are retained. Venous
return is driven across the venous resistance by the difference between the mean systemic vascular pressure upstream (largely determined by intravascular volume and venous tone) and intrathoracic vena caval pressure. For patients who make weak inspiratory efforts, mean intrathoracic pressure rises during ventilation with positive pressure, especially when positive end-expiratory pressure (PEEP) is used or auto-PEEP is generated. This raised intrapleural pressure increases intracavitary right atrial pressure (but reduces the transmural pressure), causing cardiac output to fall unless mean systemic pressure rises sufficiently to compensate (see Chapter 1). Major increases in lung volume may also compress the vena cava and increase resistance in the region of the diaphragm. Initiating ventilatory support is most likely to compromise forward cardiac output in patients with depleted intravascular volume who fail to make breathing efforts. The effect may be quite different in patients with congestive failure in whom relief of the ventilatory burden, in combination with the reduction of left ventricular afterload and reduced transvascular pressures that positive intrathoracic pressure provides, may dramatically improve forward output and reduce pulmonary vascular engorgement. (Conversely, resumption of a high ventilatory workload increases O2 demands and lowers intrathoracic pressure sufficiently to seriously destabilize the ischemic or failing heart.) Impairment of cardiac output is particularly likely to occur in patients whose mean intrathoracic pressures rise is the highest (e.g., those with chest wall restriction, good lung compliance, and air trapping) and in patients who are volume depleted, β-blocked, or unable to venoconstrict adequately. Profound deterioration may occur in patients developing auto-PEEP immediately after the institution of positive-pressure ventilation. Unlike hemorrhage and other states of volume depletion, compensatory heart rate responses to the lower output and blood pressure associated with positive pressure are often blunted. Usually, adverse reactions are most evident shortly after mechanical ventilation or PEEP is instituted, as slowly adapting compensatory changes in intravascular volume and vessel tone may attenuate these effects over time.
return is driven across the venous resistance by the difference between the mean systemic vascular pressure upstream (largely determined by intravascular volume and venous tone) and intrathoracic vena caval pressure. For patients who make weak inspiratory efforts, mean intrathoracic pressure rises during ventilation with positive pressure, especially when positive end-expiratory pressure (PEEP) is used or auto-PEEP is generated. This raised intrapleural pressure increases intracavitary right atrial pressure (but reduces the transmural pressure), causing cardiac output to fall unless mean systemic pressure rises sufficiently to compensate (see Chapter 1). Major increases in lung volume may also compress the vena cava and increase resistance in the region of the diaphragm. Initiating ventilatory support is most likely to compromise forward cardiac output in patients with depleted intravascular volume who fail to make breathing efforts. The effect may be quite different in patients with congestive failure in whom relief of the ventilatory burden, in combination with the reduction of left ventricular afterload and reduced transvascular pressures that positive intrathoracic pressure provides, may dramatically improve forward output and reduce pulmonary vascular engorgement. (Conversely, resumption of a high ventilatory workload increases O2 demands and lowers intrathoracic pressure sufficiently to seriously destabilize the ischemic or failing heart.) Impairment of cardiac output is particularly likely to occur in patients whose mean intrathoracic pressures rise is the highest (e.g., those with chest wall restriction, good lung compliance, and air trapping) and in patients who are volume depleted, β-blocked, or unable to venoconstrict adequately. Profound deterioration may occur in patients developing auto-PEEP immediately after the institution of positive-pressure ventilation. Unlike hemorrhage and other states of volume depletion, compensatory heart rate responses to the lower output and blood pressure associated with positive pressure are often blunted. Usually, adverse reactions are most evident shortly after mechanical ventilation or PEEP is instituted, as slowly adapting compensatory changes in intravascular volume and vessel tone may attenuate these effects over time.
Barotrauma
Pathogenesis of Alveolar Rupture
The varied forms of pulmonary barotrauma—interstitial emphysema, pneumomediastinum, pneumoperitoneum, subcutaneous emphysema, cyst formation, and pneumothorax (PTX)—are among the most troublesome iatrogenic consequences of intensive care. Although PTX may arise from such diverse medical problems as pulmonary infection or infarction, Pneumocystis pneumonia, or spontaneous or cough-induced rupture of a pleural bleb, a confined set of etiologies accounts for most PTX in the intensive care unit (ICU): pulmonary and pleural punctures, lung necrosis, and ventilator barotrauma. Projectiles, sharp instruments, and displaced rib fractures cause PTX by direct puncture of the visceral pleura. PTX may also complicate any medical procedure in which a needle enters the thorax, especially thoracentesis, pleural biopsy, transthoracic aspiration of a pulmonary mass, and central line placement. (Therefore, if a subclavian or internal jugular central line must be placed, it is prudent to select the side with an existing chest tube.) If air enters the pleural cavity inadvertently via a needle without inflicting lung injury, the gas will spontaneously reabsorb, often without the need for intervention. Discrete single punctures of the lung are less likely to cause problems than multiple punctures or slashing actions of the bevel of the needle. Disruption of the visceral pleura and necrotizing pulmonary infections may also cause PTX.
With increased awareness of the dangers of high airway pressure, ventilation-associated barotrauma now occurs much less frequently than in previous decades. Direct rupture of the visceral pleura undoubtedly occurs as a consequence of distention by positive pressure in many patients with diseased or injured lungs, but the barotrauma that complicates mechanical ventilation can also develop by a more circuitous path. Rupture of weakened alveolar tissues is particularly likely to affect “nonpartitional” or “marginal” alveoli, which have bases contiguous to relatively immobile structures— vessels, bronchioles, or fibrous septae. During positive-pressure ventilation (or severe blunt chest injury that occurs with the glottis closed), alveolar pressures rise more than interstitial pressures, allowing pressure gradients to develop between marginal alveoli and the contiguous perivascular connective tissues. If rupture occurs, extra-alveolar gas follows a pressure gradient down the path of least resistance, tracking along the perivascular sheaths toward the hilum. The interstitial emphysema produced en route may be detected against the radiopaque background of infiltrated lung as lucent streaks and small cysts that do not correspond to the bronchial anatomy. The gas continues to track centrally, forming a pneumomediastinum
that may or may not be evident on routine films (see Chapter 11).
that may or may not be evident on routine films (see Chapter 11).
In the absence of preexisting mediastinal pathology, extra-alveolar gas dissects along fascial planes, usually decompressing into the soft tissues of the neck (subcutaneous emphysema) or retroperitoneum (pneumoperitoneum). PTX occurs in a minority of such cases (perhaps 20% to 30%) when soft-tissue gas ruptures into the pleural space via an interrupted or a weakened mediastinal pleural membrane. Interstitial emphysema, pneumomediastinum, and subcutaneous emphysema have little hemodynamic significance and seldom affect gas exchange significantly in adults. Because their presence signals alveolar rupture and the potential for PTX to evolve, these signs are important to detect in the ventilated patient. Pressure gradients usually favor decompression of interstitial gas into the mediastinum. However, when normal bronchovascular channels are blocked, gas accumulates locally or migrates distally to produce subpleural air cysts that compress parenchymal vessels, create dead space, increase the ventilatory requirement, and cause major problems for ventilation-perfusion matching. The development of cystic barotrauma is an ominous finding that usually presages tension PTX a short time afterward.
Bronchopulmonary Injury
Until quite recently, the development of bronchial damage was believed to occur only rarely in adult patients. Autopsy studies of patients ventilated at moderately high pressures for extended periods, however, have demonstrated that small airways unsupported by cartilage can sustain considerable damage at high airway pressures. Airway distortion predisposes to cystic parenchymal damage, disordered gas exchange, and impaired secretion clearance.
Cystic Barotrauma
Widespread cystic barotrauma is most likely to develop in young patients with necrotizing pneumonitis, narrowed airways, and retained secretions. Alveolar rupture and focal gas trapping are key to its pathogenesis. As predicted by the law of Laplace (P = 2T/R), the pressure (P) required to maintain a fixed tension (T) in the wall of a spherical structure falls as its radius (R) increases. Therefore, it is not uncommon for a cyst created by positive airway pressure to grow quickly to a large dimension (>10 cm in diameter). Once underway, cystic barotrauma tends to be pernicious and self-reinforcing. As cysts develop, they compress normal lung tissue, stiffening the lung and increasing the airway pressure needed for effective ventilation. Furthermore, blood flow diverts away from areas of cyst expansion, creating dead space that increases the ventilatory requirement and therefore the mean alveolar pressure. Increased peak and mean airway pressures accentuate the tendency for further lung damage, whereas higher requirements for alveolar ventilation tend to keep the patient dependent on the ventilator. Secretion management, treatment of infection, and most importantly, reduction of airway pressure are fundamental to effective management.
Systemic Gas Embolism
For patients with acute respiratory distress syndrome (ARDS) ventilated with high tidal pressures and maintained with relatively low left ventricular filling pressures (pulmonary arterial “wedge pressures”), peak and mean alveolar pressures may exceed pulmonary venous pressures in certain lung regions. If alveolar rupture opens a communication pathway to the vascular system, this pressure gradient may drive air into systemic circulation. Microbubbles can then cause vasospasm, seizure, stroke, or myocardial infarction (MI). Usually, the MI is inferior, as the buoyant air percolates into the right coronary artery, which lies anteriorly and superiorly in the supine position.
Uncomplicated Pneumothorax
Ordinarily, the visceral and parietal pleural surfaces are approximated during both phases of the respiratory cycle. The negative pressure between them is maintained by the joint tendencies of the chest wall to expand and the lungs to recoil to their natural resting volumes. At equilibrium, these opposing forces create a moderately negative pleural pressure. PTX disrupts the normal relationship of the lung to the chest wall. The lung collapses toward its resting volume. Simultaneously, PTX allows the chest wall to expand toward its unstressed volume, which occurs at approximately 60% of the normal vital capacity. The natural tendency of the chest wall to expand—“the counterspringing effect”—is diminished or lost when thoracic volume increases. The gas that separates them impairs coupling between the lung and chest wall. Outward migration of the chest wall puts the bellows at a mechanical disadvantage. Expansion of the chest wall also shortens the resting length of the inspiratory muscles, placing them on a less
advantageous portion of their length-tension relationship. Less obviously, the total force developed by the muscles of the chest wall normally distributes over a larger surface area than that offered by the collapsed lung. Therefore, even if the inspiratory muscles generate the same intrapleural pressure, the total force applied to the lung is reduced in proportion to the degree of lung collapse. As tidal excursions of the unaffected lung increase to maintain ventilation, elastic and flowresistive work increase. This increase is well tolerated by healthy patients with adequate ventilatory reserve. However, those with significant airflow obstruction, neuromuscular weakness, or parenchymal restriction may experience dyspnea, progressive hypoventilation, and respiratory acidosis.
advantageous portion of their length-tension relationship. Less obviously, the total force developed by the muscles of the chest wall normally distributes over a larger surface area than that offered by the collapsed lung. Therefore, even if the inspiratory muscles generate the same intrapleural pressure, the total force applied to the lung is reduced in proportion to the degree of lung collapse. As tidal excursions of the unaffected lung increase to maintain ventilation, elastic and flowresistive work increase. This increase is well tolerated by healthy patients with adequate ventilatory reserve. However, those with significant airflow obstruction, neuromuscular weakness, or parenchymal restriction may experience dyspnea, progressive hypoventilation, and respiratory acidosis.
Tension Pneumothorax
The term tension PTX implies sustained positivity of pleural pressure. The intrapleural pressure exceeds atmospheric pressure during expiration and at times during inspiration as well. A tension component can develop when a ball valve mechanism pumps air into the pleural cavity during spontaneous breathing but occurs much more commonly during positive-pressure ventilation. Positive intrapleural pressure expands the ipsilateral chest cage, rendering the muscles less-efficient generators of inspiratory pleural pressure. A shifting mediastinum helps the affected side to accommodate to the increasing pressure but encroaches on and deforms the contralateral hemithorax, compromising lung expansion. (Parenthetically, single-lung transplantation performed in emphysematous subjects can evoke similar “tension” physiology.) Eventually, rising pleural and central venous pressures impede venous return sufficiently to cause hemodynamic deterioration. It should be emphasized that tension can develop without lung collapse or even major volume loss (e.g., when the lung is heavily infiltrated, air trapped, or regionally bound by pleural adhesions). Vigorous inspiratory efforts tend to maintain intrapleural pressure (averaged for both lungs over the entire respiratory cycle) at nearnormal levels until the patient fatigues, is sedated, or receives increased machine assistance. Then, abrupt hemodynamic deterioration may occur as mean pleural pressure rises sharply. Such considerations explain why many patients who develop pneumothoraces while mechanically ventilated show a tension component and why ventilated patients with PTX who receive sedating or paralyzing drugs frequently undergo abrupt hemodynamic deterioration. For the nonintubated patient, muscle fatigue and respiratory arrest may precede the cardiovascular collapse described classically with the tension PTX syndrome.
Risk Factors for Barotrauma
Although the peak airway cycling pressure has been cited frequently as the most important risk factor for ventilator-related barotrauma, it clearly is not the only one (Table 8-1). In fact, magnitude of tidal pressure may be overwhelmed by other cofactors. The correlation between airway pressure or PEEP and barotrauma is not a tight one. A necrotizing parenchymal process, inhomogeneous lung pathology, young age, excessive airway secretions, and duration of positive-pressure ventilation are major predispositions. The process of alveolar rupture is one that seems to require sustained hyperexpansion of fragile alveoli. Therefore, the mean alveolar pressure, averaged over an entire respiratory cycle, may be an important contributing factor. As major determinants of peak and mean alveolar pressures, minute ventilation requirement and high levels of PEEP contribute to the PTX hazard. (PEEP itself without high inflation pressure contributes little to the risk of barotrauma, especially if applied within the range over which lung recruitment is its primary action.) Peak dynamic (PD) and static (PS) airway pressures (plateau) seem to contribute most to the multivariate risk equation. Peak dynamic airway pressure can be reduced by improving lung compliance, reducing tidal volume (VT) or PEEP, lowering airflow resistance, or slowing peak inspiratory flow rate. On first consideration, it might seem that PS (the pressure that acts in conjunction with thoracic compliance to determine overall lung volume and alveolar stretch) should correlate even more closely with PTX than PD. However, although PS does bear a strong relationship to PTX, airway resistance varies greatly among the bronchial channels of a nonhomogeneously affected lung, so that
increasing the dynamic pressure within the central airway may encourage regional overdistention and alveolar rupture in channels with open pathways to weakened alveoli. Ball valving may also occur. Therefore, raising the peak flow rate is not risk free. On the other hand, slowing the rate of inspiratory flow prolongs alveolar distention, increasing the mean alveolar pressure. This is true especially for patients with severe airflow obstruction. Improving airway resistance or lung compliance and reducing alveolar pressure by lowering VT and/or PEEP are preferable methods for lowering PD.
increasing the dynamic pressure within the central airway may encourage regional overdistention and alveolar rupture in channels with open pathways to weakened alveoli. Ball valving may also occur. Therefore, raising the peak flow rate is not risk free. On the other hand, slowing the rate of inspiratory flow prolongs alveolar distention, increasing the mean alveolar pressure. This is true especially for patients with severe airflow obstruction. Improving airway resistance or lung compliance and reducing alveolar pressure by lowering VT and/or PEEP are preferable methods for lowering PD.
TABLE 8-1 PREDISPOSITIONS TO BAROTRAUMA | ||||||||
---|---|---|---|---|---|---|---|---|
|
There does not seem to be a sharp threshold value of peak ventilator cycling pressure below which lung rupture fails to occur. As a rule, however, PTX becomes much more likely at peak ventilator cycling pressures greater than 40 cm H2O. A peak tidal pressure greater than 35 cm H2O usually achieves or exceeds the alveolar volume corresponding to total lung capacity in a patient with a normal chest wall. Conversely, when the chest wall is stiff, high plateau pressures may be well tolerated. Secretion accumulation, blood clots, or foreign objects can increase the degree of nonhomogeneity or create ball-valve phenomena that increase the barotraumas hazard. The crucial roles of mechanical and structural nonhomogeneity may explain why PTX tends to develop 1 to 3 weeks after diffuse lung injury, a time when some regions are healing while others remain actively inflamed.
Diagnosis of Barotrauma
Clinical Features of Pneumothorax
Early recognition of PTX is of paramount importance for patients ventilated with positive pressure because of their proclivity to develop tension. During episodes of acute clinical deterioration compatible with PTX, the mortality risk rises when physicians delay intervention, awaiting roentgenographic confirmation. Pleuritic chest pain, dyspnea, and anxiety comprise the most common symptoms of uncomplicated PTX. Symptoms indicative of other forms of extraalveolar air that may precede PTX include transient precordial chest discomfort, neck pain, dysphagia, and abdominal pain. These nonspecific symptoms often are transient. Tension PTX frequently provokes tachypnea, respiratory distress, tachycardia, diaphoresis, cyanosis, or agitation. For patients receiving volume-cycled ventilation, the airway manometer usually (but not always) shows increased peak inspiratory (and peak static or plateau) airway pressures as PTX develops, especially if tension is present. Compliance of the respiratory system usually falls from previous values. During volume cycling, the ventilator may “pressure limit” or “pop off,” resulting in ineffective ventilation. During pressure-controlled ventilation, a decreased tidal volume and/or minute ventilation may be the only clue to increasing ventilatory impedance.
Close examination of the affected hemithorax often reveals signs of hyperexpansion with unilateral hyperresonance, tracheal deviation, diminished ventilatory excursion, and reduced breath sounds on the affected side. The examination must be performed carefully, as massive atelectasis can present a similar clinical picture, simulating PTX on the contralateral side. Auscultation and percussion are essential. Massive gas trapping and auto-PEEP is another effective mimic of PTX, especially if hyperinflation or infiltration is distributed asymmetrically. Palpation of the cervical tissues and suprasternal notch is important to detect subcutaneous emphysema or a trachea deviated away from the side of tension. Tension is reflected in elevations of central venous, right atrial, and pulmonary arterial pressures. Such hemodynamic changes generally do not occur during atelectasis.
Radiographic Signs of Barotrauma
Extra-Alveolar Gas
Extra-alveolar air in the lung parenchyma can manifest as interstitial emphysema or as subpleural air cysts. Both are easiest to detect when the parenchyma is densely infiltrated. Sharp black lines that outline the heart, great vessels, trachea, inferior pulmonary ligament, or diaphragm suggest mediastinal emphysema, even when the pleural membrane itself cannot be visualized (Fig. 8-1). The “complete diaphragm” sign indicates that the heart is separated from the diaphragm by a cushion of air. Subcutaneous emphysema, subdiaphragmatic air, and pneumoperitoneum are other manifestations of barotrauma that may precede or coexist with PTX. Subpleural air cysts commonly are often seen in basilar regions.
Pneumothorax
A number of radiographic signs of PTX deserve emphasis (see Chapter 11). A smooth, two-sided visceral pleural line is diagnostic but must be distinguished from a skin fold and other artifacts at the body surface. The magnification feature is often needed if the lung is otherwise normal, especially
when digitized films are viewed from a remote monitor. (By providing an air-tissue contrast, infiltrates make the job of PTX detection much easier.) Even when pulmonary infiltrates are extensive, a pleural line may be particularly difficult to detect on a standard supine view if pleural air loculates anteriorly or if ribs or mediastinal vessels obscure the pleural margin. Two useful markers of occult PTX visible on supine films are the “deep sulcus” sign and hyperlucency centered over the ipsilateral abdominal upper quadrant. For bedridden patients, a lateral decubitus view allows air to collect along the upper margin of the hemithorax, facilitating visualization. An expiratory chest radiograph also may prove revealing. (Although the volume of intrapleural air remains constant, it occupies a greater percentage of the available thoracic volume.) PTX under tension can be suspected strongly from a single film when diaphragmatic inversion or extreme mediastinal shift occurs. A sequence of films demonstrating progressive migration of the mediastinal contents into the contralateral hemithorax indicates that the diagnosis was delayed but confirms its validity. Life-threatening tension PTX can exist without complete lung collapse or mediastinal displacement if a portion of the lung adheres to the pleura, if the lung is densely infiltrated, if the airway is obstructed, or if the mediastinum is immobilized by infection, fibrosis, neoplasm, or previous surgery. The mere presence of a chest tube may not prevent tension from developing if the tube is nonfunctional or inadequate to evacuate a large air leak, if the pocket drained is loculated, if the drainage holes are within the major fissure, or if intraparenchymal tension cysts coexist. In fact, by indicating the presence of and tendency for tissue rupture, the presence of a chest tube should heighten suspicion for an inapparent PTX on the same side.
when digitized films are viewed from a remote monitor. (By providing an air-tissue contrast, infiltrates make the job of PTX detection much easier.) Even when pulmonary infiltrates are extensive, a pleural line may be particularly difficult to detect on a standard supine view if pleural air loculates anteriorly or if ribs or mediastinal vessels obscure the pleural margin. Two useful markers of occult PTX visible on supine films are the “deep sulcus” sign and hyperlucency centered over the ipsilateral abdominal upper quadrant. For bedridden patients, a lateral decubitus view allows air to collect along the upper margin of the hemithorax, facilitating visualization. An expiratory chest radiograph also may prove revealing. (Although the volume of intrapleural air remains constant, it occupies a greater percentage of the available thoracic volume.) PTX under tension can be suspected strongly from a single film when diaphragmatic inversion or extreme mediastinal shift occurs. A sequence of films demonstrating progressive migration of the mediastinal contents into the contralateral hemithorax indicates that the diagnosis was delayed but confirms its validity. Life-threatening tension PTX can exist without complete lung collapse or mediastinal displacement if a portion of the lung adheres to the pleura, if the lung is densely infiltrated, if the airway is obstructed, or if the mediastinum is immobilized by infection, fibrosis, neoplasm, or previous surgery. The mere presence of a chest tube may not prevent tension from developing if the tube is nonfunctional or inadequate to evacuate a large air leak, if the pocket drained is loculated, if the drainage holes are within the major fissure, or if intraparenchymal tension cysts coexist. In fact, by indicating the presence of and tendency for tissue rupture, the presence of a chest tube should heighten suspicion for an inapparent PTX on the same side.
Value of the Computed Tomography Scan
The thoracic computed tomography (CT) scan is an invaluable aid in determining whether lucency represents parenchymal or pleural air. In fact, accurate placement of a chest tube into a loculated pocket of gas or fluid may require insertion under direct CT guidance. When doubt exists regarding the effectiveness of the chest tube in draining gas or fluid and/or the exact placement of the tube, CT performed with the tube connected to an appropriate level of suction is currently the “gold standard.” Modern CT scanning equipment can acquire a volumetric (helical or spiral) data set very quickly (within seconds). Reconstruction of a two-dimensional image can then be initiated along sagittal and coronal as well as the traditional axial plane. Indeed, highly informative three-dimensional reconstruction is now being applied with increasing frequency, imaging that usually leaves little doubt regarding the relevant anatomy.
Management of Pneumothorax and Pleural Effusion
General Principles
Pulmonary barotrauma developing in the setting of acute lung injury is a self-perpetuating, autoamplifying, and potentially lethal process that must be prevented. After extra-alveolar air begins to manifest in its cystic form, impaired gas exchange often forces an increase in minute ventilation requirement and, therefore, in mean airway pressure. In turn, higher mean airway pressure may worsen the tendency for alveolar rupture. Key principles for avoiding barotrauma (Table 8-2) include (a) treat the underlying disease, especially suppurative processes; (b) maintain excellent bronchial hygiene but minimize unnecessary coughing; (c) reduce the minute ventilation requirement by limiting agitation, fever, metabolic acidosis, and bronchospasm; and (d) reduce peak and mean airway pressures by limiting PEEP and tidal volume, by permitting hypercapnia, and by increasing the percentage of
spontaneous versus machine-aided breaths. During volume-cycled ventilation, reducing tidal volume modestly (e.g., by 100 to 300 mL) may greatly reduce PD and PS. Peak flow should be set to the lowest value that satisfies inspiratory demand without incurring additional patient work or auto-PEEP, thus lowering peak dynamic (but not peak static or mean alveolar) pressure. Although several modes of ventilation have been advocated to reduce peak airway pressure (high frequency, pressure supported, and pressure controlled ventilation), their therapeutic efficacy in preventing barotrauma is unproven.
spontaneous versus machine-aided breaths. During volume-cycled ventilation, reducing tidal volume modestly (e.g., by 100 to 300 mL) may greatly reduce PD and PS. Peak flow should be set to the lowest value that satisfies inspiratory demand without incurring additional patient work or auto-PEEP, thus lowering peak dynamic (but not peak static or mean alveolar) pressure. Although several modes of ventilation have been advocated to reduce peak airway pressure (high frequency, pressure supported, and pressure controlled ventilation), their therapeutic efficacy in preventing barotrauma is unproven.
TABLE 8-2 PREVENTING VENTILATOR-RELATED LUNG RUPTURE | ||||||||
---|---|---|---|---|---|---|---|---|
|
Chest Tube Drainage
Indications for Thoracostomy
Vigilant observation and conservative management are appropriate options in the spontaneously breathing, uncompromised patient with a small PTX. If the patient is breathing spontaneously, simple observation with close radiographic follow-up is often a reasonable strategy for well-compensated patients who experience a small PTX without symptoms after thoracentesis, aspiration needle biopsy, or central line placement. Many such patients will not require more aggressive management. Serial radiographs (or CTs) must demonstrate gradual improvement. (The same is not often true for patients receiving positive pressure ventilation, especially is ventilating pressures are high.) Although spontaneous resolution of PTX is a slow process, an air collection that fails to show convincing improvement over several days may indicate an ongoing leak, with equilibration between the rates of leakage and absorption. After leakage stops, the absorption of intrapleural air occurs at a variable rate (see Chapter 11), averaging approximately (original %)/(1.5%) each day. Even a moderate PTX may take weeks to resolve. (For example, a 15% PTX would be expected to reabsorb completely in 15/1.5 = 10 days.) During this period, the partially collapsed lung may clear secretions poorly. Because large collections of undrained pleural air predisposes to infection of the lung or pleural space, hypoxemia, and proteinaceous pleural fluid collections, it must be evacuated by catheter aspiration or chest tube. The high risk of tension mandates early decompression in the mechanically ventilated patient. Other patients to consider for early intervention are those with ipsilateral pneumonia or secretion retention, ventilatory insufficiency, or high ventilation requirements.
Tube Options
The ideal chest tube system provides a reliable, low-impedance conduit that ensures efficient, unidirectional evacuation of gas and liquid from the chest. It should produce subatmospheric intrapleural pressure and reapproximate the lung to the chest wall. To be in best position for drainage of unloculated air, the tube should be directed superiorly and anteriorly. Small tubes can be introduced anteriorly in the second intercostal space, but for reasons of relative comfort or cosmetics, are often placed laterally. Tubes to be placed in loculated pockets are best inserted by an interventional radiologist. Large tubes are best introduced laterally in the midaxillary line of the sixth-to-seventh interspace and are directed upward, taking care to avoid entry into a fissure. Starting from a lower interspace, the operator should begin far enough posteriorly to avoid subdiaphragmatic placement. When the PTX is distributed evenly (unloculated) and suction is used, the actual position of the tube tip makes little difference. However, if loculations develop, a poorly placed chest tube, especially one not connected to suction, may fail to evacuate the appropriate region. Although large tubes usually are needed to drain substantial collections of fluid, chest tubes placed for simple air drainage are usually 28 French in caliber or smaller. Iatrogenic pneumothoraces without major air leak often can be managed in stable patients with short flexible tubes of very small diameter (usually pigtail catheters). These can be attached to a drainage system (with or without suction) or to a lightweight flutter valve (Heimlich valve) to facilitate ambulation. Such small catheters can be introduced easily but sometimes entail considerable discomfort, especially in young patients. Larger tubes are selected if substantial liquid drainage is also needed. Tube radius is a major determinant of the evacuation capability of the system. However, unless the tube caliber is very small, the leak is very large, or the drainage system is compromised by
fibrin or debris, system resistance usually does not limit the rate of evacuation. (Tube placement and patency are much more important.)
fibrin or debris, system resistance usually does not limit the rate of evacuation. (Tube placement and patency are much more important.)
Drainage Apparatus
One-way drainage usually is ensured by a water seal (Fig. 8-2). In recent years, lightweight, self-contained, integrated function, disposable plastic units are used almost exclusively. A collection chamber (or “bottle,” a label used in deference to the original equipment serving the same function) is inserted in tandem and proximal to the water seal column. The water seal chamber is separated from the collection chamber because accumulated liquid drainage would make air leakage difficult to visualize and, more importantly, would create sufficient back pressure to hinder lung expansion as fluid accumulates. Regulation of the suction pressure applied to the chest tube, a function once done by a water-filled and continuously bubbling “suction regulating” chamber, is now accomplished by a simple noise-free mechanical or needle valve.
Monitoring Tube Function
Fluid movements in the water seal tube reflect tidal variations of intrapleural pressure adjacent to the tube. For the spontaneously breathing patient, fluid rises in inspiration and falls during exhalation. The reverse is true during passive inflation with intermittent positive pressure. The direction of tidal fluctuations during triggered machine cycles varies with the vigor of the respiratory effort. Without suction applied, fluctuations of liquid within a dependent loop of drainage tubing or of the water seal (“tidaling”) can provide useful clinical information. (Tidal fluctuations are smaller during suction.) An abrupt increase in tidaling magnitude suggests undrained air surrounding the tube, lobar atelectasis, upper airway obstruction, impaired secretion clearance, or hyperpnea. Decreased tidaling can reflect resolution of any of these problems, partial obstruction of drainage, or decreased air leakage through a bronchopleural fistula (BPF). Absent fluctuations may be explained by tube obstruction with fibrin, blood clots, or extrinsic compression. Because of the risks of infection, the chest tube should be removed as soon as it no longer fulfills a useful function. Because 25 to 100 mL of liquid will form each day
within the normal pleural space, drainage of this amount is expected through a functioning tube that has full access to the pleural space. (Tubes draining loculated spaces may be patent despite lesser output.) If the tube is patent, noticeable fluctuations within the water seal chamber should occur during moderate respiratory efforts.
within the normal pleural space, drainage of this amount is expected through a functioning tube that has full access to the pleural space. (Tubes draining loculated spaces may be patent despite lesser output.) If the tube is patent, noticeable fluctuations within the water seal chamber should occur during moderate respiratory efforts.
A “dead tube” (<50 mL/24 h of drainage, no gas leak, and no respiratory fluctuation) should either be made functional or pulled. A tube that drains empyema, blood, or thick fluid can often be maintained patent by periodic “stripping.” Quite often, a clogged or ineffective tube can be reopened at least transiently by sterile injection of an intrapleural fibrinolytic (e.g., alteplase or tissue plasminogen activator, TPA), followed by a brief period of clamping. When a complicated effusion (without ongoing air leak) requires drainage, injecting 25 to 50 mg of 100 mL alteplase in approximately 100 mL of saline into the pleural pocket and letting it act behind a chest tube clamped for several hours may break up loculations and reestablish effective drainage. This process may need repeating multiple times over several days. Although experience has shown that intrapleural bleeding and/or alteration of the systemic coagulation profile rarely occur, TPA instillation is not entirely without risk. A tube that clogs repeatedly presents a genuine risk of infection and probably requires extraction (“pulling”). Another tube or more aggressive surgical approach, such as video-assisted thoracoscopic debridement, should then be considered if the need for draining additional drainage is still apparent. If the water seal level rises (toward the patient) and ceases to fluctuate with respiration after several days of declining drainage, pleural reapposition probably has occurred, and the tube should be removed after radiographic confirmation. The rising level reflects sealing of the air leak and subsequent absorption of the air contained within the chest tube.
Persistent bubbling at the water seal signals an air leak within lung, tubing, or connections. If the leak is within the lung, its magnitude can be quantified during volume-cycled mechanical ventilation by comparing the set inspiratory volume delivered by the machine to the recovered exhaled tidal volume (corrected for the circuit tubing component). (Some drainage systems also provide crude airflow detectors.) If the inspired and expired volumes are nearly equivalent, air leakage is likely to originate external to the lung. Cessation of the air leak when the tube is clamped near the chest wall indicates a BPF or air entry at the incision site. The latter can be excluded by careful approximation of the skin edges and the application of airtight occlusive dressings. If the leak does not stop after clamping near the chest wall, there has been a breach of drainage system integrity. Migratory (transient) clamping of the tubing (moving away from the patient) will then allow more precise localization. Each connection should be carefully inspected.
Suction
Indications for Suction
Natural pressure gradients (fluid siphon effects, expiratory contractions, inspiratory development of positive pressure during mechanical ventilation) are often adequate to empty the pleural space of gas and liquid. However, suction will generally be needed for large air leaks or for drainage of viscous effusions or blood. When the lung is entirely surrounded by gas, pressure applied to one portion of the pleural surface distributes equally throughout the hemithorax. However, when normal pleural surfaces are approximated, the negative pressure applied to one area transmits poorly to other regions. The explanation is that lung tissues adjacent to the tube effectively isolate the pocket of negative pressure. In addition, the tissues may be drawn into the “eyes” of the tube, preventing general transmission of applied negative pressure. When this happens, increasing suction only increases the risk for local tissue injury. For similar reasons, tubes placed inadvertently into a major or minor fissure are surrounded by very pliable lung surfaces and tend to drain poorly—but this is not invariably true. Adhesions with loculation also may impede pressure transmission—negative or positive. Massive amounts of fluid or air can collect and tension can develop in sectors remote from a functioning but isolated tube. In this instance, multiple tubes in different locations may be required. Unless the draining fluid is unusually viscid, suction usually can be discontinued (but the water seal maintained) when gas bubbling stops.
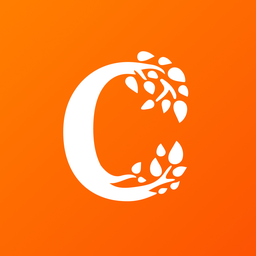
Full access? Get Clinical Tree
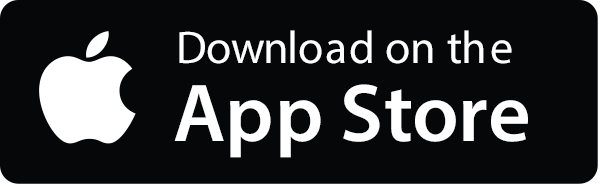
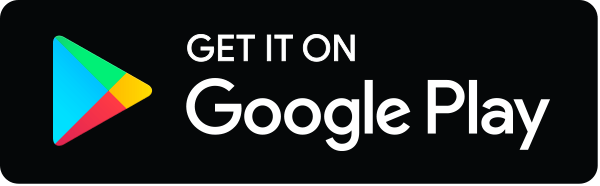
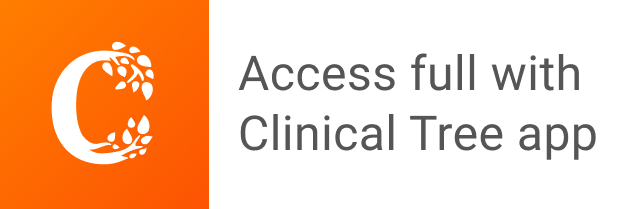