Mu receptor
Kappa receptor
Delta receptor
Molecular details and endogenous agonists
7- transmembrane domain G-protein
Enkephalins (high affinity)
Beta endorphin (high affinity)
Dynorphins (low affinity)
Subtypes: μ(mu) 1, 2 and 3
7- transmembrane domain G-protein
Dynorphins
Subtypes: κ(kappa) 1, 2 and 3
7- transmembrane domain G-protein
Enkephalins
Beta endorphin
Subtypes: δ(delta) 1 and 2
Clinical effects
μ(mu) 1: Analgesia
μ(mu) 2:
Respiratory depression
Ileus/constipation
Pruritus
μ(mu) 3:????
Analgesia
Dysphoria
Halluciantions
Reward/aversion
Decrease in locomotor
Activity and arousal
Analgesia
Seizures
Increase in locomotor
Activity
Respiratory depression
Ileus
Agonists
Morphine, codeine, hydromorphone
Fentanyl, alfentanil, sufentanil, remifentanil
Tramadol
Meperidiine, methadone
Ketamine
Ketazocine
Terpenoid
Pentazocine
Butopharnol
Nalorphine
Nalguphine
Norbuprenorphine
Cannabidiol
Tetrahydrocannabinol
Antagonist
Naloxone, naltrexone, methylnaltrexone,
Nalbuphine
Naloxone, naltrexone
Buprenorphine
Trazodone
Naltrindole
Agonist
/antagonist
Buprenorphine
Buprenorphine
7.2.1 Mu Opioid Receptors (MOR)
MOR is the site of action of the endogenous peptides enkephalins, β(beta)-endorphin, dynorphin A, nociceptins, and agonists such as morphine, fentanyl congeners, methadone, and meperidine. The human MOR gene (OPRM1) spans over 200 kb and consists of 11 exons that combine to yield 17 splice variants [4, 5]. More than 700 single nucleotide polymorphisms have been described in the gene with variations that differ between races and ethnicities [5]. More clinically relevant is the fact that genetic variations in the OPRM1 gene can explain differences in the analgesic response to opioids between individuals [5, 6]. Thus, heterozygous patients with MOR A118G and G1947 mutations show less opioid consumption after surgery and homozygous carriers of both A118G and 158Met COMT allelic variants also require less morphine than their heterozygous counterparts [7–9].
MORs are located in the central nervous system (CNS) and in peripheral tissues of the body. Centrally, MORs are found in the periaqueductal gray (PAG) region of the brainstem and medial thalamus. MORs located at spinal level (superficial dorsal horn) also play a role in opioid-induced analgesia [10, 11]. Peripheral MORs have been found in leukocytes, myenteric cells, pulmonary neuroendoncrine and pancreatic cells, skeletal muscle, and nerve terminals located in the lung, joints, and blood vessels such as the portal vein [12].
The MOR belongs to the seven-transmembrane receptor superfamily. It is coupled with Gi/o proteins and as expected its activation is responsible for short- and long-term effects on neuronal and non-neuronal cells [13]. After binding of MOR agonists to the receptor, the release of the inhibitory Gi protein causes inhibition of adenylate cyclase, reduces cyclic 3 ′, 5 ′ adenosine monophosphate (cAMP), and reduces levels of protein kinase A activation [14]. MORs also modulate the activity of the G0 protein, which inhibits Ca2+ and activates K+ channels [13]. The net result of activation of MORs is a decrease in the release of neurotransmitters (presynaptic) and hyperpolarization of membrane potentials (pre- and post-synaptic). MORs also have the ability to form dimers (homo or heterodimerization), which can also explain the differences in the analgesic response between subjects. In fact, the formation of heterodimers can alter agonist or antagonist selectivity, or switch intracellular signaling at the level of G proteins [15].
There are at least 2 well-described MOR subtypes receptors: mu1 (MOR1) and mu2 (MOR2). The analgesic effect of MOR agonists is caused by the binding to the MOR1 subtype receptor while their action on the MOR2 is responsible for most of the adverse effects related to opioids (respiratory depression, sedation, pruritus, prolactin release, dependence, anorexia, and sedation) [16, 17]. Interaction between the serotonergic and opioidergic systems also helps to explain the analgesic effects of opioids [18]. Acute morphine administration appears to enhance the synthesis, release, and metabolism of serotonin—especially in projection areas of the dorsal raphe nucleus and median raphe nucleus; in contrast, chronic exposure to morphine decreases release of 5-HT from the nerve terminals [18–20]. Furthermore, interaction with other receptors such as the dopaminergic (D1) receptors has been demonstrated in neurons of the cortex and caudate nucleus [21].
The orphan G protein-coupled receptor (GPCR) Opioid Receptor (NOP) is the most recently discovered opioid receptor [22]. Activation of the NOP receptor in supraspinal pain pathways (PAG) has hyperalgesic effects while its activation at the level of spinal structures appears to induce analgesia [23]. NOP receptors are insensitive to opioid agonists and antagonists such as morphine and naloxone. Noceptin/Orphanin FQ (N/OFQ) is the natural ligand peptide for the NOP receptor and does not show cross-reactivity with MORs. It is worth mentioning that the intrathecal administration of morphine and nociception has demonstrated supra-additive effects.
7.2.2 Classes of Opioids
There are 4 chemical classes of opioids: phenanthrenes, benzomorphans, phenylpiperidines, and diphenylheptanes. Morphine, codeine, hydromorphone, levorphanol, oxycodone, hydrocodone, oxymorphone, buprenorphine, nalbuphine, and butorphanol belong to the phenanthrenes class. Pentazocine is a member of the benzomorphans class, and propoxyphene and methadone are classified in the diphenylheptanes class. Lastly, the phenylpiperidines include fentanyl, alfentanil, remifentanil, sufentanil, and meperidine. On the basis of their pharmacodynamics profiles, opioid analgesics can be classified as full agonists (i.e., morphine, fentanyl or remifentanil) or agonists-antagonists (i.e., buprenorphine, pentazocine and nalbuphine) [24]. Opioid agonists-antagonists can show high affinity for MOR but exhibit little efficacy [24].
7.3 Pharmacokinetics and Pharmacodynamics
Regardless of the route of administration, opioids need to cross the blood-brain barrier (BBB) in order to cause analgesia. The 2 main mechanisms of transport are passive diffusion and active transport, with the former being the most predominant method (◘ Table 7.2 [25–44]). Passive transport depends on the concentration of the drug, hydrophilicity, lipophilicity, and the degree of hydrogen bonding of the drug. It is generally accepted that hydrophilic opioids, such as morphine, will tend to accumulate in tissues with high contents of water and the opposite will occur with lipophilic drugs, such as fentanyl. Although passive diffusion of opioids across the BBB is accepted as the main kinetic mechanism of action, it cannot fully explain the kinetics of opioids in the CNS. For instance, the transport of morphine can be modulated by ATP-binding cassette (ABC) transporters such as P-glycoprotein (P-gp), whose expression appears to be decreased after long-term exposure to that opioid. Furthermore, the poor penetration of morphine has been linked to its active efflux from the brain to the blood by the P-gp at the BBB [45]. Therefore, passive and active mechanisms of transport govern the rate of diffusion of opioids across the BBB.
Recommended dose | Alfentanil | Remifentanil | Fentanyl | Sufentanil | Hydromorphone | Morphine | Methadone |
---|---|---|---|---|---|---|---|
Intravenous Bolus Maintenance | 20–75 μ(mu)g/kg 0.2–1.5 μ(mu)g/kg/min | 0.5–1 μ(mu)g/kg 0.05–0.5 μ(mu)g/kg/min | 1–3 μ(mu)g/kg 0.5–2 μ(mu)g/kg/h | 0.1–1 μ(mu)g/kg 0.1–1 μ(mu)g/kg/h | 5–10 μ(mu)g/kg | 0.05–1 mg/kg | 5–10 mg |
Epidural Bolus Infusion | 250–400 μ(mu)g 400 μ(mu)g/h | _____ _____ | 0.5–1 μ(mu)g/kg 5–20 μ(mu)g/h | 0.1–0.3 μ(mu)g/kg 1–6 μ(mu)g/h | 5–10 μ(mu)g/kg 5–40 μ(mu)g/h | 30–100 μ(mu)g/kg 200–400 μ(mu)g/h | 5–6 mg 0.5 mg/h |
Spinal Bolus Infusion | _____ _____ | _____ _____ | 10–50 μ(mu)g | 5–7.5 μ(mu)g | 5–40 μ(mu)g | 30–200 μ(mu)g | |
Alfentanil | _____ | _____ | A:F 10:1 | _____ | _____ | _____ | _____ |
Remifentanil | A:R 16–70:1 | _____ | R:F 1:1 | _____ | _____ | _____ | _____ |
Fentanyl | _____ | _____ | _____ | S:F 5–10:1 | M:F 50–100:1 | 2–5:1 | |
Sufentanil | _____ | _____ | _____ | _____ | _____ | M:S 1000:1 | _____ |
Hydromorphone | _____ | _____ | H:F 5–10:1 | _____ | M:F 4–7:1 | _____ | |
Morphine | _____ | _____ | _____ | _____ | _____ | _____ | _____ |
Methadone | _____ | _____ | _____ | _____ | _____ | _____ | _____ |
Morphine, one of the most commonly used opioids, is a full MOR agonist with poor liposolubility, low plasma protein binding (35%), and limited blood-brain barrier penetration [46, 47]. Hydromorphone, also a full agonist of MOR, is a semi-synthetic hydrogenated ketone derivative of morphine with a potency that is 7–10 times higher than its precursor [48]. Protein binding of hydromorphone is low (◘ Table 7.3) [49–55]. Fentanyl, a synthetic phenylpiperidine with high affinity for the MOR, is 80–100 times more potent than morphine; therefore, it is considered a strong opioid. Fentanyl has a high liposolubility, good penetration of the BBB, and is highly bound to proteins (84%) [56]. Alfentanil is an agonist of the MOR that has one-third to one-fourth of the potency of fentanyl. Alfentanil has a higher protein binding (79%) than fentanyl [56, 57]. Sufentanil is a thienyl analog of fentanyl with high liposolubility and a potency that is 3–5 and 5–10 times higher than fentanyl when given epidurally or intravenously, respectively [56]. Sufentanil is very highly protein bound (92.5%) [56]. Remifentanil is a 4-anilidopiperidine derivative of fentanyl and is an ultra-short-acting μ(mu)-opioid receptor agonist [58]. Meperidine is the least potent of the phenypiperidine class. In fact, it has approximately 10% of the effectiveness of morphine; however, some of its analgesic effects are related to its local anesthetic properties. Meperidine is not highly protein bound (58–75%) [59]. Methadone is a synthetic MOR agonist and an antagonist of the NMDA receptor. Methadone is formulated as a racemic mixture of 2 enantiomers; the R form binds to MOR, while S configuration acts as the NMDA antagonist. The S isomer also inhibits reuptake of serotonin and norepinephrine. Methadone is 60–90% bound to plasma proteins, mostly the acid α(alpha)1-globulins [60]. Tramadol is considered a weak MOR agonist. Tramadol has 1/10 of the potency of intravenous morphine [61]. It is formulated as a racemate, where the (+)-enantiomer stimulates MOR and inhibits reuptake of 5-HT, whereas the (−)-enantiomer inhibits noradrenaline reuptake [62]. Tramadol is 20% plasma protein bound [63]. Propoxyphene is a weak synthetic analgesic with a similar structure to methadone and an equipotency similar to codeine. Some of the analgesic effects of propoxyphene can be explained by its antagonistic actions on the NMDA receptor; however, its use has been discouraged because of significant adverse effects [64]. Tapentadol is a novel MOR agonist and inhibitor of norepinephrine uptake. Tapentadol is 2–3 times less potent than morphine and has shown low protein binding. Cebranopadol is a newly developed nociceptin receptor (NOP) agonist that has also cross-reactivity with MORs, δ(delata), and κ(kappa) receptors. In rodents, cebranopadol is 180–4800 times more potent than morphine and has shown analgesic actions in inflammatory, neuropathic, and bone cancer pain [65, 66]. Cebranopadol is not available for clinical use in humans.
Morphine | Hydromorphone | Alfentanil | Fentanyl | Sufentanil | Remifentanil | |
---|---|---|---|---|---|---|
Vd (l/kg) | 0.95–2.9 | 1.22–2.9 | 0.4–1 | 3–8 | 3–5 | 0.1–0.4 |
Clearence (mL/min) | 482 ± 502 | 1660 | 139 ± 88 | 498 ± 190 | 552.8 ± 314.7 | 4117–4950 |
Terminal elimination t 1/2 (min) | 78 ± 21 | 112–204 | 108 ± 81 | 68 ± 33 | 59.2 ± 22 | 11.8 ± 5.1 |
Context-sensitive half-life | 50–55 | > 100 | 20–30 | 3–5.4 | ||
Protein binding | 35% | 14% | 79% | 84% | 92.5% | 92% |
Analgesic onset (min) | 7.5 | 10 | 0.75 | 1.5 | 1 | 1 |
7.3.1 Intravenous Opioids
The intravenous injection of morphine, hydromorphone, fentanyl, sufentanil, alfentanil and remifentanil follow a 3-compartment pharmacokinetic model [67–76]. For most opioids, the 3 compartments are:
- 1.
The systemic blood after immediate injection
- 2.
Tissues with good blood flow such as liver and brain
- 3.
Tissues with slow and/or low blood flow such as fat and muscles
Equilibration between tissues and blood occurs faster in compartment 2 than in 3. Extensive tissue uptake and high liposolubility in the third compartment (reservoir) are factors that affect the elimination time of lipophilic drugs such as fentanyl and sufentanil. Subsequently, the return of these drugs from fat tissues to the blood is a rate-limiting step for their ultimate clearance from the body [71]. For example, there is less tissue accumulation of alfentanil, in comparison to fentanyl, because it has a lower protein-binding capacity and a lower lipid solubility [73]. Methadone and tramadol have a 2-compartment model such that after a bolus injection they are followed by an exponential decay [77, 78].
The onset of action of opioids is highly dependent on lipid solubility and equilibrium between the blood and brain. Thus, sufentanil and fentanyl (both are very lipophilic) have a short onset of action while drugs such as hydromorphone (hydromorphone) have an onset of approximately 5 min with a peak effect 20 min later. This is due to delayed penetration of the blood-brain barrier [79]. The fastest onsets of action are for alfentanil and remifentanil (approximately 1–2 min).
The offset of action of opioids is also variable. The context-sensitive half-time (defined as the time necessary to achieve a 50% decrease in blood or plasma concentration after termination of a variable-length, continuous infusion targeted to maintain a steady-state concentration, where the “context” is the duration of the infusion) of remifentanil is 2–3 min and independent of the duration of infusion [80]. For procedures lasting longer than 10 min, alfentanil has a longer duration of action than remifentanil but it is one-third of that of fentanyl [81]. Furthermore, in comparison to sufentanil and fentanyl, alfentanil has a shorter elimination rate that makes accumulation after repeated or sustained administration considerably lower [57, 71]. Therefore, the recovery from remifentanil bolus is faster than the recovery from alfentanil, which in turn is quicker than from a bolus of sufentanil and fentanyl [55, 82]. For long infusions of these opioids, it is expected that remifentanil would have the fastest recovery and alfentanil and sufentanil should produce a faster recovery than fentanyl [55, 81, 82].
Morphine has a longer time to maximum analgesia in comparison to hydromorphone. This is due to the slow increase in the active metabolite morphine-6-glucuronide that contributes significantly to the analgesic effects of morphine. The duration of action of hydromorphone is at least 120 min while that for morphine has been estimated between 180 and 240 min [79]. Methadone’s duration of action is 4–21 h with a half-life of 12–150 h [77, 83]. The analgesic effect of tramadol is 3–4 h [84].
The volume of distribution (Vd) is another important pharmacokinetic parameter. It is affected by factors such as age or comorbidities and fluid status of the patients (Vd may be increased by renal failure and liver failure and decreased in dehydration). Furthermore, the Vd is usually larger in obese than non-obese patients. The Vd of morphine and hydromorphone are similar (0.95–2.9 l/kg versus 1.22–2.9 l/kg, respectively) but relatively smaller than that of methadone (3.3–6 l/kg) [85–89]. Sufentanil and fentanyl have a total volume of distribution (Vd) of 3–5 l/kg and approximately 4 l/kg, respectively; in contrast, remifentanil and alfentanil have smaller Vd approximately 0.190 l/kg and 0.86–1.03 l/kg, respectively [57, 68, 71, 90]. Of note, the Vd of remifentanil is closely related to lean body weight rather than total body weight [70, 91]. Meperidine and tramadol have similar volume of distribution that ranges from 3.5 to 6 l/kg [76, 92, 93].
7.3.2 Epidural and Intrathecal Opioids
When opioids are injected into the epidural space, passive diffusion and active transport mechanisms deliver the drug across the BBB and into the brain. These were the same mechanisms as opioids injected via the intravenous route [94]. However, concentrations in the lumbar cerebrospinal fluid (CSF) of a liposoluble opioid such as fentanyl and sufentanil after their intravenous administration are significantly lower or undetectable compared to those measured in the CSF after epidural injection [94].
The epidural space deserves special consideration because it can act as a reservoir of liposoluble drugs. Liposoluble opioids such as fentanyl and sufentanil have longer epidural residence times and terminal elimination half times compared to less liposoluble drugs such as morphine [94, 95]. This explains why morphine achieves higher concentrations in the cerebrospinal fluid than sufentanil and fentanyl; hydrophilic drugs move preferentially to the CSF after single epidural injections and lipophilic drugs tend to remain in the epidural space. During prolonged infusion of lipophilic opioids in the epidural, the plasma concentrations of these drugs are similar to that of an intravenous infusion [96]. The use of vasoconstrictors such as epinephrine (2 μ[mu]g/ml) can reduce the rate of systemic absorption of opioids and improve the quality and duration of analgesia [97, 98].
Epidurally injected drugs cross through the meninges via simple diffusion. This passive mechanism involves 2 steps: first the movement of the drug across the cell membrane (lipid bilayer) of arachnoid cells, and then across intra- and extracellular fluids [94]. Lipophilic opioids, such as fentanyl or sufentanil, will accomplish the first step with ease but the second step with some difficulty; the opposite can be expected for hydrosoluble drugs such as morphine or hydromorphone [99].
When opioids are injected intrathecally, they also reach receptors located in the spinal cord by diffusion. The factors that govern the kinetics of intrathecal opioids are baricity, temperature, volume, speed of administration, and lipid solubility [99, 100]. Lipid solubility has a significant impact on the speed of onset, duration of action, clearance, and the volume of distribution (Vd) of the drug in the CSF; thus, lipophilic opioids show quicker onset and a Vd larger than drugs such as morphine. The clearance of sufentanil in cerebrospinal fluid is orders of magnitude greater than intrathecal morphine [101, 102]. Moreover, the terminal half-life of sufentanil is reported as 0.6–1.4 h, and that one for morphine is 3.1 h [101, 102]. Spread of intrathecal opioids appears to be inversely related to lipid solubility. This explains why morphine shows significant rostral spread after intrathecal administration compared to sufentanil or fentanyl, which provide a more segmental distribution (analgesia). Lastly, opioids move out of the intrathecal space by diffusion into blood vessels; however, the different clearance rates between opioids contribute to the overall duration of analgesia [100]. For instance, an opioid with a low clearance rate such as morphine produces significantly longer analgesia than sufentanil or alfentanil. In contrast, fentanyl rapidly distributes out of the CSF by diffusing into the epidural space and epidural fat [94]. The concentrations of opioids in plasma after single intrathecal administration are negligible. This fact guides the use of opioids in spinal analgesia during labor as fetuses are exposed to minimal amounts of plasma narcotics.
7.3.3 Metabolism and Excretion
The major metabolic pathway for most opioids is oxidation. The exceptions are morphine and hydromorphone, which primarily undergo glucuronidation, and remifentanil, which is cleared by ester hydrolysis. Factors affecting liver metabolism—such as cigarette smoking, age, sex, obesity, and use of medications—can modify the activity of metabolizing enzymes [103]. Morphine undergoes metabolism in the liver by uridine diphospho glucuronosyltransferases (UGT) 2B7 (primarily), UGT1A3, CYP3A4, and CYP2C8 into several metabolites. The most important of them are morphine-3-glucuronide (M3G) and morphine-6-glucoronide (M6G), which are excreted by the kidneys [87, 104, 105]. Approximately 10% of morphine is excreted as unchanged morphine [106]. M3G has no analgesic activity but it is a neuroexcitatory metabolite. In contrast, M6G and normorphine do have analgesic activity. Although the affinity of M6G for the MOR2 subtype is lower than that for morphine, M6G can accumulate in the presence of renal failure and may cause respiratory depression [87, 106–108]. Liver disease can also alter the pharmacokinetics of morphine. Higher plasma concentrations (Cmax) of the glucuronide metabolites have been reported in patients with non-alcoholic steatohepatitis [109]. Thus, the duration of action or side effects associated with morphine might be increased in patients with moderate to severe liver impairment [110]..
Hydromorphone also undergoes liver metabolism but in contrast to morphine, it does not appear to have a 6-glucuronide metabolite. Instead, it is extensively metabolized to a hydromorphone-3-glucuronide, dihydroisomorphine glucuronide, dihydromorphine, hydromorphone-3-sulfate, norhydromorphone, and nordihydroisomorphine [111]. Of all these metabolites, hydromorphone-3-glucuronide deserves attention because it has neuroexcitatory effects more potent than those observed with M3G [112, 113].
Fentanyl is metabolized by the liver by cytochrome P450 (CYP) 3A4 to active and inactive metabolites [67, 114]. Hepatic impairment may lead to accumulation and prolonged action of fentanyl. Around 10% of the drug undergoes renal excretion [67, 114]. Sufentanil is metabolized in the liver by N-dealkylation or O-demethylation [115, 116]. The desmethyl metabolite has 10% of the activity of sufentanil while the other metabolites are inactive [116]. Since the metabolites are excreted in urine and feces, desmethyl sufentanil can accumulate in patients with renal failure after repeated or continuous administration. The kinetics of sufentanil are not significantly altered in cirrhotic patients [90]. Alfentanil is metabolized and eliminated by the liver through O-dealkylation and N-dealkylation as the main metabolic pathways [56, 116]. The metabolites of alfentanil have no pharmacologic activity. However, in patients with reduced liver function, prolonged effects can be expected after a large single or cumulative dose of alfentanil because of its hepatic-dependent elimination and increased unbound fraction [56, 116]. Remifentanil is rapidly metabolized via extrahepatic, nonspecific blood and tissue esterases to remifentanil acid (inactive). Because it is not a substrate for plasma cholinesterase, its metabolism is not subject to genetic variance. Remifentanil acid is primarily excreted by the kidneys [69]. The pharmacokinetics of remifentanil are not significantly affected after short periods of administration in patients with renal or liver impairment; however, the time to offset and metabolic ratio might be prolonged or increased after prolonged infusions (>72 h) or large doses [68, 117, 118].
Meperidine is metabolized by the liver into meperidinic acid (hydrolysis) and normeperidine (N-demethylation), which is then hydrolyzed to normeperidinic acid [119]. Excretion of normeperidine and normeperidinic acid is primarily renal [76]. Normeperidine has significant excitatory effects in the CNS. Prolonged administration of meperidine and in patients with renal impairment can lead to neurological derangements [120, 121].
Methadone is metabolized in the liver and intestines and excreted by the kidney (15–60%) and in feces (20–40%), an advantage in patients with renal insufficiency or failure. CYP3A4 (N-methylation) is the main mechanism in methadone metabolism (inducible) [116, 122]. Tramadol undergoes hepatic metabolism via the cytochromes P450 (CYP2D6). There are 5 known active and inactive metabolites [123]. O-demethyltramadol (M1) is an active metabolite with 200 times the μ(mu)-affinity of tramadol. The other 4 metabolites are mono-N-de-methyltramadol (M2), N,N- didemethyltramadol (M3), N,N,O-tridemethyl-tramadol (M4), and N,O-deme thyltramadol (M5). All metabolites undergo conjugation with glucuronic acid and sulfate before excretion by the kidney [63, 124]. Tapentadol is highly metabolized in the liver to largely inactive metabolites that are excreted by the kidneys [125].
7.3.4 Effect on Circulation
Most opioids reduce sympathetic tone and enhance vagal and parasympathetic tone in a dose-dependent manner [126–128]. Fentanyl analogs can produce arterial hypotension by a centrally mediated reduction in systemic vascular resistance and direct vasodilatory effects [129]. For instance, the administration of sufentanil and alfentanil for surgical analgesia is associated with minimal cardiovascular depression or alteration of the activity of the autonomic nervous system; however, in large doses, they can cause severe hypotension even in healthy subjects and especially in patients with poor cardiovascular reserve [130, 131]. Although rare, profound bradycardia or even asystole can also be observed after the administration of fentanyl analogs, especially in conjunction with vagal stimulating effects of laryngoscopy or during pediatric strabismus surgery (oculocardiac reflex) [132–134]. Electrophysiological studies in humans show that fentanyl and remifentanil provoke a dose-dependent depressor effect on sinus and AV node function [135, 136]. The administration of methadone has been associated with prolongation of the QT interval and torsades de point on the electrocardiogram. However, this phenomenon appears to be dose-dependent and in the presence of other drugs that affect the QTc [137, 138]. This adverse event has led health authorities to issue a black box warning on the use of methadone.
Meperidine, morphine, and to a lesser extent hydromorphone can cause histamine release and subsequently hypotension and myocardial depression [129, 139]. Meperidine has atropine-like effects on heart rate; hence, tachycardia can be observed. Because fentanyl and its derivates cause minimal or no release of histamine, they provide better hemodynamic stability than morphine [80].
7.3.5 Effect on Respiration
The effects of opioids on respiration include: (1) reduction in minute ventilation by decreased respiratory rate, tidal volume or both; (2) decreased response to hypoxia; and (3) depression of the CO2 response (chemosensitivity) [140]. As a result, the apneic threshold and resting end-tidal PCO2 are increased by opioids. The depressant effects of opioids are centrally mediated by: (1) acting on the brain stem and medulla, and (2) peripherally on the carotid bodies [140, 141]. The respiratory pattern also changes after opioid administration—in particular in opioid naïve patients. Irregular or periodic breathing, apneic periods (respiratory pausing), and expiratory delays are often seen after the administration, of even low doses, of opioids.
The ventilation response to hypoxia is also reduced sometimes to a greater extent than the depression to hypercapnia [142]. Activation of the MOR mediates most of the effects of opioids on the respiratory system; however, delta receptors may also exert some inhibitory effects on respiration [143, 144]. Kappa receptors appear to be devoid of respiratory depressant activity [145].
The depression of the respiratory drive is dose-dependent and also proportional to the analgesic potency [140]. Thus, after fentanyl administration, CO2 response curves usually showed combined decreases in slope and displacements to the right that do not return to baseline levels for more than 1 h. An earlier return to normal levels is seen with alfentanil, which is a less potent narcotic compared to fentanyl.
7.3.6 Effect on Other Organs
As expected, opioids can modulate the activity of the CNS, which can be measured by electroencephalography (EEG) and evoked potentials (EP). The predominant effect is a generalized slowing of the spontaneous EEG. Morphine increases the activity of alpha and beta bands and tends to raise the delta band activity over time [81, 148, 149]. The effect of intravenous or intrathecal opioids is minimal on evoked potentials [150–153].
Opioids have significant effects on the endocrine system. They cause inhibition of each level of the hypothalamic-pituitary-gonadal axis (HPG). In the hypothalamus, opioids decrease the secretion of GnRH. The production of LH is reduced when opioids bind to MOR in the pituitary gland [154]. Opioids also interfere with the function of the ovaries and testes, which have been linked to a reduction in the production of sex hormones [155]. Opioids also interfere with the release and function of several hormones of the hypothalamus-pituitary-adrenal (HPA) axis [156]. The overall effect is suppression of the axis—a termed also known as “opioid-induced adrenal insufficiency.” The magnitude of HPG and HPA axis suppression is not the same among different opioids; therefore, opioid rotation has been recommended in patients with opioid-induced hypogonadism or adrenal insufficiency.
7.3.7 Side Effects and Toxicity
Respiratory Depression
Opioids can induce respiratory depression when administered systemically or neuraxial [157, 158]. The incidence of respiratory depression is 0.01–7% [159, 160]. Respiratory depression is dose-dependent and commonly observed after the co-administration of other CNS depressant (i.e., general anesthesia, magnesium sulfate, or diphenhydramine) [161, 162]. Advanced age, obstructive sleep apnea, obesity and intrathecal use of opioids are risk factors for respiratory depression [162–165]. When given neuraxial, early and late respiratory depression can be observed with highly and poorly lipid soluble drugs, respectively. Fentanyl or sufentanil can depress the respiratory system within 20–25 min after the spinal administration. Intrathecal morphine-induced respiratory depression has been described as biphasic with an early phase (10–90 min) secondary to vascular absorption and a late phase due to rostral spread (6–18 h) [158, 166].
Pruritus
Pruritus is a common side effect after the administration of opioids. Itching is often located on the face; however, it also can be described as a “whole body itch.” The mechanism involves interactions between MOR and estrogen receptors or MOR1 and serotoninergic (5-HT3) receptors located in the medulla and the dorsal horn of the spinal cord [167]. Pruritus can occur after the systemic or neuraxial administration of opioids, although the incidence is the highest after intrathecal injections. The incidence and duration of pruritus appear greater and longer with the use of epidural morphine than with hydromorphone and fentanyl [168].
Nausea and Vomiting
Nausea and vomiting are also side effects observed after the administration of opioids. In the general surgical population the incidence ranges from 20% to 80% [169]. Opioids can induce nausea and vomiting through several mechanisms including: (1) direct effects on the chemoreceptor trigger zone (CTZ), specifically in the area postrema or in the vomiting center in the brainstem; (2) enhanced vestibular sensitivity; and (3) delayed gastric emptying. Direct activation of CTZ MOR appears to be the main mechanism of opioid-induced nausea and vomiting. The delta receptor as well as interaction with other “emetogenic” receptors such dopamine-2 (D2), histamine-1 (H1), 5-HT3 receptor, acetylcholine (ACh), tachykinin NK1 (NK-1), and cannabinoid receptor-1 (CB1) might also be involved in the pathophysiology. It has been proposed that once CTZ MOR and delta-opioid receptors are activated, they initiate signaling to the vomiting center via dopamine D2 receptors and 5-HT3 receptors, which explain the efficacy of anti-sertoninergic and anti-dopaminergic drugs [170].
Urinary Retention
The incidence of urinary retention after opioid administration ranges from 0% to 80% depending on the type of opioid and the patient population (the elderly are at greater risk). The effects of intrathecal or epidural opioids on bladder function are potency- and dose- dependent. Long-acting opioids are associated with the highest rate of urinary retention [173]. Opioids induce urinary retention by primarily acting on supraspinal and spinal MOR, even when administered systemically. Activation of MOR located in the PAG area of the midbrain and Barrington’s nucleus inhibits reflex bladder contractions [174, 175]. MORs present in the spinal cord participate in urinary retention via inhibition of the micturition [176]. The intrathecal administration of delta and kappa opioid receptor agonists produces little or no effect on volume-evoked bladder contractions [174].
Opioid-Induced Bowel Dysfunction
Constipation is the most common side effect associated with the chronic administration of opioids. It can be observed after a short period of administration as well. All 3 main opioid receptors are present in the gastrointestinal (GI) tract; however, the MOR mediates most of the central effects [177]. MOR activation induces hyperpolarization of enteric neurons that results in a decrease in rhythmic gut contractions and mucosal secretions [178, 179]. Recent studies indicate that opioid-induced activation of toll-like receptor 4 (TLR-4) may also play a role in opioid-induced bowel dysfunction since antagonism of the TLR-4 receptor appears to stimulate peristalsis after morphine administration [180].
The effects of opioids agonists in the GI tract are not homogeneous. Rectal muscle fibers appear to be more sensitive to exogenous opioids than proximal colonic fibers, which can explain why administration of these drugs results in intestinal ileus and predominantly dry stools [181].
Cognitive Dysfunction and Neuromuscular Effects
Somnolence, memory impairment, and cognitive disorders can be observed even after a single dose of opioids [182]. These side effects are dose-dependent and reversed by the administration of MOR antagonists [183]. Drugs such as morphine and hydromorphone can also trigger delirium via the excitatory effects of their 3-glucoronide metabolites. Similarly, normeperidine (an active metabolite of meperidine) can cause cognitive impairment by its known anticholinergic effect.
Chest rigidity (wooden chest syndrome) can occur during induction of anesthesia with lipophilic synthetic opioids such as fentanyl, sufentanil, and remifentanil [184]. This side effect is dose-dependent, and also observed after rapid intravenous injections in particular in newborns or elderly patients. The mechanism remains poorly understood; however, activation of central MOR and decreased dopaminergic activity in the nucleus raphe pontis and the caudate nucleus has been implicated mechanistically [185–187].
Opioid-Induced Hyperalgesia (OIH)
OIH is defined as a condition in which patients requiring chronic opioid therapy develop enhanced pain sensitization. The clinical diagnosis of OIH can be a challenge [188]. OIH is suspected when there is a change in the characteristics of the preexisting pain syndrome from well localized to a more generalized and ill-defined pain along with increased requirements in the consumption of opioids [188, 189]. Patients with OIH can also present as an exaggeration of preexisting painful disorder. OIH also has been described after short-term exposure to opioids as it occurs during the perioperative surgical period [188, 189]. In the surgical setting, high doses of fentanyl and remifentanil have been implicated in the development of OIH [190, 191].
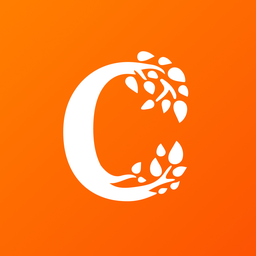
Full access? Get Clinical Tree
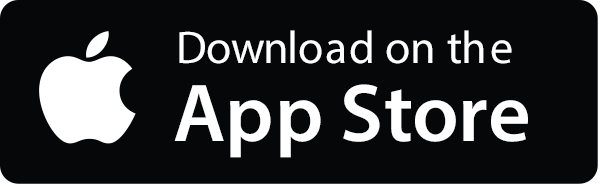
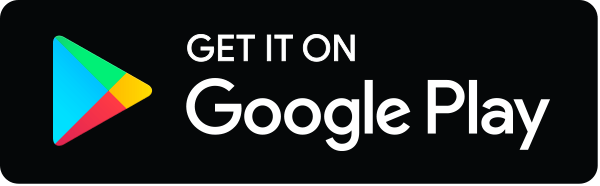