When selecting an anesthetic, anesthetists often consider, among others, the questions presented in Table 1–1. To answer these questions, anesthetists turn to textbooks, journal articles, and drug package inserts. These resources provide dosing recommendations (ie, bolus doses and infusion rates) and important features of anesthetic drugs but often fall short of providing useful answers. Anesthetists therefore rely on years of training and experience to formulate the correct dose and safely administer it. Experienced anesthetists develop a sense of how individual drugs behave and can easily tailor them to meet the needs of their patients. For example, anesthetists have a good “feel” for what a 3-mL (150 mcg) intravenous bolus of fentanyl will accomplish in a healthy adult and can accurately predict the onset and duration of analgesic effect.
What drug or drug combination will work best for the patient? |
What are the adverse effects? |
After giving a dose, when will it start to have an effect and how long will it last? |
When using a combined technique, how do different anesthetics interact with one another to prolong various drug effects? |
How do age, body habitus, blood loss, gender, organ function, medications, health supplements, and so on, influence the onset and duration of anesthetic effects? |
Once turned off, how long will it take for the patient to emerge from anesthesia? |
In procedures associated with moderate to severe postoperative pain, what dose of analgesic will be safe but still provide adequate pain control? |
Most anesthetics, however, are neither single agents nor are they consistently administered to healthy patients. Suppose an anesthetist has to answer the same questions posed in Table 1–1 for that 3-mL bolus of fentanyl in the presence of 2% sevoflurane. How are the onset and duration changed? For a morbidly obese patient, how does the difference in body habitus influence the onset and duration of effect? With unanticipated severe blood loss, how will fentanyl behave?
The most widely used predictor of anesthetic effect is the minimum alveolar concentration (MAC), the concentration of inhalation agent in the alveoli necessary to keep 50% patients from moving when exposed to a noxious stimulus. Originally used in laboratory investigations to differentiate the potency of inhalation agents from one another, MAC has become a well-known clinical descriptor of drug effect. In fact, modern physiologic monitors display estimates of anesthetic effect as percentages of MAC based on expired concentrations of inhalation agents.
MAC and its derivatives (the concentration of inhalation agent in the alveoli necessary to block an autonomic response in 50% patients when exposed a noxious stimulus [MACbar] and the concentration of inhalation agent in the alveoli in which 50% patients are awake [MACawake]) are by design not reflective of what anesthetists aim to achieve—why blunt the response to a noxious stimulus in only 50% of patients? Why not 99% or 100%? In practice, clinicians use percentage multiples of MAC along with opioids and other anesthetics to ensure that all of their patients are adequately anesthetized. Although this approach works, it remains somewhat vague. For example, what multiple of MAC (or MACbar) combined with what dose of opioid are required to ensure 99% of patients do not move during skin incision or are unconscious?
A more refined approach is to consider an anesthetic as a composite of effects. This is consistent with how anesthesia is delivered—a combination of sedative hypnotics, analgesics, and neuromuscular blockers. An ideal anesthetic technique would tailor doses to achieve a 95% or 99% probability of each effect. An ideal monitor system would have an easily measured parameter for each effect. Such capability would likely help avoid overdosing, minimize hemodynamic disturbances, and plan for rapid emergence while ensuring adequate analgesia. Unfortunately, with the exception of neuromuscular blockade monitors and to some extent processed electroencephalograph (EEG) monitors, such devices do not yet exist.
With advances in clinical pharmacology and through the use of simulation, sophisticated tools have been developed that predict the onset and duration of sedation, unresponsiveness, analgesia, and neuromuscular blockade. Although not a direct measurement, simulation provides real-time visualization of the time course of anesthetic drug concentrations and their effects both for individual drugs and drug combinations. Simulations rely on complex models of pharmacokinetics, pharmacodynamics, and drug interactions to predict drug behavior. Until recently, these models have been too mathematically cumbersome and difficult to display. With advances in computer technology, they are now being introduced as educational supplements and as drug displays at the point of care (Figure 1–1). These displays create pictures of drug behavior that are useful when considering some of the questions listed in Table 1–1.
As an example, consider the simulation of an induction with propofol and fentanyl. This simulation provides predictions of propofol and fentanyl concentrations over time and a prediction of response to laryngoscopy (Figure 1–2). With this picture, several points of interest are easily appreciated. One, fentanyl takes longer to reach peak effect than propofol. Administration should be offset by 3 to 4 minutes if it is desirable to have them reach peak effect simultaneously. Two, by itself, fentanyl contributes very little to blunting the response to laryngoscopy (peak probability of < 1%). Three, propofol alone blunts the response to laryngoscopy for more than 2 minutes. Four, fentanyl and propofol combined blunt the response to laryngoscopy for more than 4 minutes. Similar pictures can be drawn for other effects of interest such as loss of responsiveness or onset of ventilatory depression.
Figure 1–2
How simulations are used to create a “picture” of drug effect. In this simulation, published pharmacokinetic1,2 and pharmacodynamic models3 are used to predict the loss of response to laryngoscopy following bolus doses of fentanyl (2 mcg/kg) and propofol (2 mg/kg). The top plot presents the effect-site concentrations for each drug over 14 minutes. The fentanyl was administered 3 minutes prior to the propofol, so that they would both peak at the same time. The middle plot presents the probability of no response to laryngoscopy for fentanyl and for the fentanyl and propofol combined. The bottom plot presents the probability of no response to propofol and for the fentanyl and propofol combined.

For selected anesthetic drugs and combinations of drug, models are well developed, making simulations easy to conduct; for others, models are not as well established. Throughout this book, where supported by high-quality research, simulations will be used to illustrate the time course of anesthetic drug behavior. For common dosing regimens, depending on available models, some or all of the concentrations and effects presented in Table 1–2 will be simulated.
Concentrations Plasma End tidal Effect site |
Effectsa (Onset and Duration) Sedation and Hypnosis Mild and moderate sedation Loss of responsiveness Electroencephalographic changesb |
Analgesia Loss of response to moderately painful stimuli (ie, electrical tetany, pressure) Loss of response to laryngoscopy and tracheal intubation Loss of response to esophageal instrumentation |
Neuromuscular Blockade Loss of train-of-four |
Ventilatory Depression |
To fully appreciate the value and limitations of simulations in a clinical context, a working knowledge of core concepts in clinical pharmacology is useful. The following sections will review how the basic elements (pharmacokinetics, biophase, and pharmacodynamics) are used to predict and illustrate drug behavior.
Pharmacokinetics describe how the body influences drug behavior; namely how drug concentrations change over time in response to a given dose. Clinical pharmacologists have developed both qualitative descriptors and kinetic models to characterize drug pharmacokinetics. Qualitative descriptors include terms such as volume of distribution, lipid solubility, percent protein binding, among many others. They provide a means of comparing drugs to one another. For example, comparing the lipid solubility of fentanyl to morphine helps explain differences in their kinetic behavior. Kinetic models use mathematical equations to empirically model how concentrations change over time. Once built, models are used to predict concentrations over time for various dosing regimens. Kinetic models are based on collecting blood samples from numerous people and compiling them to create a population kinetic model. An important limitation of kinetic models is that they are useful in illustrating the time course of drug concentration over time but do not provide any estimate of drug effect. This chapter will review both aspects of kinetics: definitions of qualitative descriptors and core concepts used in the construction of a pharmacokinetic model.
To illustrate important descriptors of drug kinetics, consider an intravenous 2-mcg/kg bolus of fentanyl (Figure 1–3). This simulation illustrates the fentanyl plasma concentration over a 20-minute period. With intravenous administration, the plasma concentration quickly rises and then wanes. During this time period, there are 3 phases.
Figure 1–3
Plasma concentrations versus time following a 2-mcg/kg bolus of fentanyl. The curve is divided into 3 phases: distribution, redistribution, and elimination. The dashed line represents the slope of the concentration curve during the elimination phase. Where this line crosses the vertical access is used to estimate the volume of distribution at steady state.

(1) The distribution phase, where fentanyl moves from the plasma into surrounding tissues. Fentanyl rapidly distributes throughout the vascular compartment and into vascular organs (the heart, brain, kidneys, and liver). At a slower rate, it distributes to muscle and at an even slower rate to adipose tissue, skin, and bone.
(2) The redistribution phase, where fentanyl returns from tissues back into the plasma. The length of drug administration influences duration of the redistribution phase. For example, if fentanyl is administered as a continuous infusion and allowed to accumulate in the muscle and adipose, once the infusion is terminated, fentanyl will continue to move back into the vascular compartment for a prolonged period of time. With fentanyl, this is especially true for infusions of duration longer than 2 hours.
(3) The elimination phase, where fentanyl is removed from the plasma.
Two general terms are used to characterize how the body influences drug behavior: volume of distribution and clearance. The volume of distribution describes how drug distributes throughout fluid and tissues in the body and is measured in terms of liters per kilogram of body weight (L/kg). Clearance describes drug removal from the body. It includes any process that leads to drug excretion or drug metabolism. The units for clearance are liters per hour (L/h).
The volume of distribution is the volume once the drug has distributed throughout the body. A simplified model of this concept is presented in Figure 1–4A. In this example, a 2-mcg/kg fentanyl bolus dose is administered to a 70-kg person with an unknown volume in their vascular compartment. After thorough mixing, the measured plasma concentration will provide an estimate of the volume of distribution using the relationship:
Figure 1–4
Schematic illustration of estimating the volume of distribution for fentanyl. A, Simplified model: a known amount of fentanyl (light blue circles indicating 140 mcg in a syringe) is administered to a 70-kg person with an unknown vascular volume. After thorough mixing, the measured concentration is 28 ng/mL, corresponding to a distribution volume of 5 L. B, Sophisticated model: a known amount of fentanyl is administered (light blue circles), and most drug diffuses into tissues outside the vascular compartment (dark blue circles). The majority of the fentanyl that remains in the vascular compartment is protein bound (brown–light blue circles). After thorough mixing, the measured fentanyl concentrations are low (0.5 ng/mL), corresponding to a distribution volume of 280 L.


Rearranging:
If the measured concentration is 28 ng/mL, then the volume of distribution would be 5 L. Unfortunately, this simplified model has significant limitations. As seen in Figure 1–3, the concentration does not stay fixed (as it would in a container) but rather declines over time. To estimate the volume of distribution under these circumstances, clinical pharmacologists extrapolate from the elimination phase of the plasma concentration versus time data as illustrated in Figure 1–3. The place where the dotted line crosses the vertical axis (at time 0) represents the fentanyl concentration (0.5 ng/mL) at which it has distributed through out the entire body and is at steady state with the plasma. Using Eqn. 2, the apparent volume of distribution is 280 L. This is significantly larger than 5 L (or an entire human for that matter)! It is important to recognize that the apparent volume of distribution does not have an anatomic correlate; it does not represent the vascular volume, the intracellular volume, or the total body water. It is only a mathematical estimate of how much fentanyl is taken up by the body.
To explain this phenomenon, consider Figure 1–4B. The vascular compartment is permeable to small molecules (including fentanyl). Substances (ie, plasma proteins) within the blood and tissues outside of the vascular compartment tightly bind to fentanyl. With much of the fentanyl diffused out of the vascular compartment and bound up in tissue, the vascular compartment fentanyl concentration is much lower, yielding an almost unbelievably large apparent volume of distribution. This phenomenon applies to many anesthetic drugs (Table 1–3).
Anesthetic Drug | Apparent Volume of Distribution |
---|---|
Opioids | |
Fentanyl | 4.0 L/kg |
Sufentanil | 5.0 L/kg |
Remifentanil | 0.4 L/kg |
Morphine | 1.0–4.7 L/kg |
Meperidine | 3.7 L/kg |
Sedative Hypnotics | |
Propofol | 2.0 L/kg |
Etomidate | 4.5 L/kg |
Ketamine | 4.0 L/kg |
Midazolam | 1.6.L/kg |
Neuromuscular Blockade Agents | |
Rocuronium | 0.3 L/kg |
Vecuronium | 0.3 L/kg |
Pancuronium | 0.1–0.3 L/kg |
Protein binding describes the amount of drug in the plasma that is protein bound. It does not describe how much drug is bound to protein or other tissues outside the vascular compartment, but proteins capable of binding drug are prevalent throughout peripheral tissues. Plasma proteins include albumin (the most abundant), α-1 glycoprotein, and lipoproteins among others. Albumin binds primarily to acidic drugs (eg, fentanyl) whereas α-1 acid glycoprotein binds basic drugs (eg, sufentanil, alfentanil, lidocaine). When bound to protein, anesthetic drugs are pharmacologically inactive. For example, fentanyl is 80% to 85% protein bound, so only 15% is available to diffuse out of the plasma. The fraction of drug not bound to protein in plasma varies significantly for many anesthetic drugs (Table 1–4).
Anesthetic Drug | % Protein Bound |
---|---|
Opioids | |
Morphine | 30%–40% |
Meperidine | 65%–70% |
Fentanyl | 84% |
Sufentanil | 92% |
Remifentanil | 70% |
Sedative Hypnotics | |
Propofol | 95%–99% |
Etomidate | 76% |
Ketamine | 60% |
Sodium Pentothal | 80% |
Midazolam | 95% |
Neuromuscular Blockade Agents | |
Rocuronium | 50%–75% |
Vecuronium | 60% |
Pancuronium | 87% |
As is appreciated by anesthetists, the onset and duration of effect can be much different than anticipated (eg, prolonged drug effect with intermediate nondepolarizing neuromuscular blockers). Various disease states that influence protein levels can significantly impact the amount of unbound drug. Low protein states (ie, liver disease, nephrotic syndrome) increase the amount of available drug and by contrast high protein states (ie, traumatic injury, surgery) decrease the amount of available drug.
Membrane permeability describes the ability of a drug to move from blood through capillary walls to peripheral tissues. In general, membrane permeability is dependent upon 3 features of an anesthetic drug: lipid solubility, ionization, and molecular size as well as membrane thickness and the integrity of endothelial cell wall junctions.
This describes the ability of a drug to move through lipid bilayer membranes. It is characterized by a partition coefficient (also known as a partition constant). It is estimated by mixing a known amount of drug in a container with both octanol (hydrophobic) and water (hydrophilic) and then measuring how much drug is in each solute. The ratio of fentanyl in octanol to fentanyl in water is 860:1. So, it has high lipid solubility and can rapidly move from plasma to peripheral tissues. This explains in part why its apparent volume of distribution is so large; most of the fentanyl has moved out of the vascular compartment. The lipophilic properties of selected anesthetics are presented in Figure 1–5.
Drugs primarily move through a lipophilic membrane in an un-ionized state. The extent a drug is un-ionized is a function of its pKa at physiologic pH. The pKa values for selected anesthetics are presented in Table 1–5. Drugs that are weak acids with a pKa more than 7.5 are almost entirely un-ionized at a physiologic pH and easily move through membranes. If the pKa is lower, then their movement through membranes becomes pH dependent. Weak bases with a pKa less than 5 are also are almost entirely un-ionized. If the pKa is higher, then movement through membranes is also pH dependent. Fentanyl is a weak base with a pKa of 8.4, so at physiologic pH, most of it exists in a un-ionized state and easily moves through tissue membranes. The amount of unbound ionized drug, depending on the pKa, can change with changes in pH (Table 1–5).
Drug Name | pKa | Percent Un-ionized | |||
---|---|---|---|---|---|
pH | |||||
7.0 | 7.2 | 7.4 | 7.6 | ||
Acids | |||||
ASA | 3.0 | 0 | 0 | 0 | 0 |
Thiopental | 7.4 | 72 | 61 | 50 | 39 |
Bases | |||||
Meperidine | 8.6 | 2 | 4 | 6 | 9 |
Fentanyl | 8.4 | 4 | 6 | 9 | 14 |
Bupivacaine | 8.1 | 7 | 11 | 17 | 24 |
Sufentanil | 8.0 | 9 | 14 | 20 | 28 |
Morphine | 7.9 | 11 | 17 | 24 | 33 |
Lidocaine | 7.9 | 11 | 17 | 24 | 33 |
Ketamine | 7.5 | 24 | 33 | 44 | 56 |
Remifentanil | 7.1 | 44 | 56 | 67 | 76 |
Midazolam | 6.1 | 89 | 93 | 95 | 97 |
Etomidate | 4.5 | 100 | 100 | 100 | 100 |
Diazepam | 3.3 | 100 | 100 | 100 | 100 |
Larger molecules are less likely to move through membranes than smaller ones. Most anesthetic drugs are small molecules and move relatively easily through membranes if un-ionized. Anesthetic drugs bound to plasma protein behave as large molecules and do not easily pass through lipid bilayers.
In general, the thicker the bilipid layer membrane, the lower the permeability. Endothelial cell wall membranes range in thickness from 0.005 to 0.01 μm. In addition to membrane thickness, the integrity of the junction between endothelial cells influences drug diffusion from plasma to peripheral tissues. Endothelial cells in the central nervous system have enhanced “tight junctions” that impede drug movement from plasma to the brain constituting the blood-brain barrier. Tight junctions consist of proteins that tightly glue cell walls together and projections from astrocytes, known as “astrocyte feet,” surround the junctions between endothelial cells.
Enantiomers are assorted structures (isoforms) of a drug. All isoforms have the identical chemical formula. The difference in enantiomers is the orientation of atoms within the drug molecule. Several anesthetic drugs exist as enantiomers. Various schemes have been used to describe how isoforms differ from one another. In some cases, 2 isomers of a molecule exist as mirror image of one another. These isoforms are characterized by their ability to rotate polarized light: clockwise (dextro, abbreviated D) versus counterclockwise (levo, abbreviated L).
More recently, drugs with several isoforms are characterized by their chirality. Chirality refers to the orientation of atoms or groups of atoms (known as substituents) about a single atom, such as carbon, that has 4 potentially asymmetric bonding sites. Substituents are prioritized based on their anatomic number (eg, size). If the smallest substituent is pointed away, the orientation of the remaining 3 substituents from smallest to largest determines the chirality as either clockwise (R for rectus) or counterclockwise (S for sinister). A given molecule may have more than 1 chiral center and more than 2 isomers. In some instances, these 2 classifications are mixed together. S(–) bupivacaine has a “S” chiral center that polarizes light in a “–” counterclockwise direction. A third method used to describe different isomers is cis and trans. This nomenclature is primarily used to describe the orientation of substituents about a double carbon bond that is unable to rotate. Table 1–6 presents a list of selected enantiomers of common anesthetic agents.
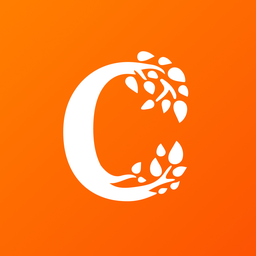
Full access? Get Clinical Tree
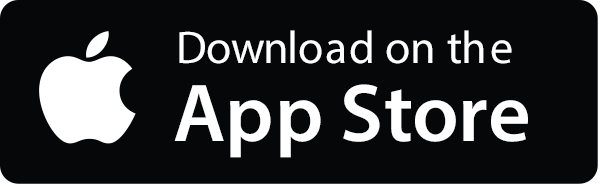
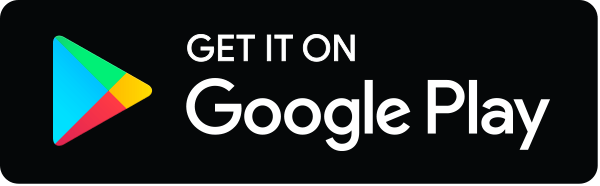