Chapter 163 The first organophosphate insecticide, triethyl pyrophosphate, was synthesized in 1859 but did not replace nicotine as a pesticide until World War II. After World War II, these compounds were used as chemical warfare agents, as organophosphorus and carbamate insecticides, and as medicinal agents. With concern about the long half-life of the organochlorine dichlorodiphenyltrichloroethane (DDT), causing it to accumulate in the environment, the organophosphate insecticides became the most common pesticides for home and industrial use. Since the increased awareness of terrorism in the 1990s, nerve agents have gained prominence as weapons of mass destruction.1 Organophosphorus pesticides work by persistently inhibiting the enzyme acetylcholinesterase, the enzymatic deactivator of the ubiquitous neurotransmitter acetylcholine. Because of the global penetration of organophosphorus compounds, inhibition occurs at tissue sites (true acetylcholinesterase and represented by erythrocyte or red blood cell [RBC] cholinesterase) and in plasma (circulating pseudocholinesterase).2 Inhibition of cholinesterase results in the accumulation and subsequent prolonged effect of acetylcholine at a variety of neurotransmitter receptors, including sympathetic and parasympathetic ganglionic nicotinic sites, postganglionic cholinergic sympathetic and parasympathetic muscarinic sites, skeletal muscle nicotinic sites, and central nervous system sites (Fig. 163-1). The accumulation of acetylcholine results in the classic SLUDGE cholinergic syndrome, manifested by hyperactivity of cholinergic responses at the receptor sites indicated previously. The clinical syndrome of muscarinic acetylcholinesterase inhibition (Table 163-1) causes postganglionic acetylcholine-induced hollow end-organ general hypersecretion, resulting in clinical findings that include miotic pupils, lacrimation, rhinorrhea, sialorrhea, bronchorrhea, vomiting, diarrhea, and urinary incontinence. Bradycardia is a classic sign of the cholinergic syndrome, but the increased release of norepinephrine from postganglionic sympathetic neurons precipitated by excess cholinergic activity at sympathetic ganglia may result in normal or even tachycardic heart rates (nicotinic effect). Sympathetic hyperactivity can cause diffuse diaphoresis, although this response is mediated by cholinergic receptors at preganglionic (nicotinic) and postganglionic (muscarinic) sites. The most lethal components of acetylcholinesterase inhibition occur in the brain and neuromuscular junction. A combination of sympathetic stimulation, involvement of the N-methyl-D-aspartate (NMDA) receptor, and enhanced acetylcholine concentrations can induce seizures.3 At the neuromuscular junction, excess acetylcholine causes hyperstimulation of the muscles with secondary paralysis, and when the diaphragm is affected, cholinesterase poisoning leads to respiratory arrest.4 Table 163-1 Nicotinic hyperstimulation of skeletal muscle leads to significant morbidity and mortality associated with acetylcholinesterase inhibitor toxicity. Signs of skeletal muscle hyperactivity include involuntary twitches, fasciculations, and hyperactive reflexes. Muscle hyperactivity eventually progresses to muscle fatigue and paralysis, including the respiratory musculature and particularly the diaphragm.4 Respiratory insufficiency may be delayed and result in death if it is not anticipated and corrected by mechanical or pharmacologic means. Acetylcholinesterase inhibitors produce direct toxic effects on the central nervous system, leading to neurologic signs of confusion, combativeness, seizures, and coma. Status epilepticus may occur in severely poisoned patients. Structural central nervous system damage may occur if seizures are not terminated rapidly.5 After acute exposures, the plasma cholinesterase levels decrease first, followed by decreases in RBC cholinesterase levels. The RBC cholinesterase level correlates best with activity at the nerve terminal.2 Patients with chronic exposures may have a normal plasma cholinesterase level but have reduced RBC cholinesterase activity, which will confirm the poisoning. The true reflection of depressed cholinesterase activity is found in the RBC activity, and even a mild acute exposure may result in severe clinical poisoning. The RBC cholinesterase level recovers at a rate of 1% per day in untreated patients and takes approximately 6 to 12 weeks to normalize, whereas plasma cholinesterase levels may recover in 4 to 6 weeks. Other laboratory studies should focus on the evaluation of pulmonary, cardiovascular, and renal function and fluid and electrolyte balance. Patients presenting with no acidosis or only a metabolic acidosis on the arterial blood gas analysis have mortality lower than that of patients with a respiratory or mixed acidosis.6 Decontamination should start in the out-of-hospital phase to prevent further absorption and subsequent toxicity and to protect care providers. Because dermal exposure is most likely, removal and destruction of clothing and thorough flushing of exposed skin may limit absorption and subsequent toxicity. Alternatively, dermal decontamination can be done with dry agents, such as military resins, flour, sand, or bentonite. Caregivers are at risk for contamination from splashes or handling of contaminated clothing. Treating personnel may be rotated to limit their exposure to the organophosphates.4 Caregivers should use universal precautions, including eye shields, protective clothing, and nitrile or butyl rubber gloves. In the case of ingestion, gastrointestinal decontamination procedures are of questionable benefit because of the rapid absorption of these compounds. Profuse vomiting and diarrhea are seen early in ingestion and may limit7 or negate any beneficial effect of additional gastrointestinal decontamination.8,9 The administration of activated charcoal after ingestion has no proven benefit in these poisonings.10 Because death is due to airway and respiratory failure, supportive care should be directed primarily toward airway management and include suctioning of secretions and vomitus, oxygenation, and, when necessary, ventilatory support. Succinylcholine can be used for intubation but may have an extremely prolonged duration (up to 3 to 7 hours) as it is metabolized by acetylcholinesterase, which is inhibited in this setting.2 It is preferable to use a competitive neuromuscular blocking agent, such as rocuronium, for rapid sequence intubation in these patients, but increased dosing may be necessary. Although some authors have advocated the use of beta-blockers to control tachycardia, this may increase cardiovascular instability and worsen bronchospasm.8,11 Most cardiovascular effects from organophosphates rarely require specific therapy. The definitive treatment of acetylcholinesterase inhibition starts with atropine. A competitive inhibitor of acetylcholine at muscarinic receptor sites, atropine reverses the clinical effects of cholinergic excess at parasympathetic end-organs and sweat glands. Large doses of atropine may be required.12 Data suggest that the more rapid the atropinization, the faster control is obtained.8,9 Suggested dosing is 1 or 2 mg of atropine (0.02-0.05 mg/kg) intravenously, with doubling of each subsequent dose every 5 minutes until there is control of mucous membrane hypersecretion and the airway clears.4,8,9,13 If intravenous access is not immediately available, atropine may be administered intramuscularly. Patients may require 200 to 500 mg of atropine intravenously during the first hour, followed by prolonged continuous infusions of 5 to 100 mg/hr to maintain adequate secretion control.13 Tachycardia and mydriasis may occur at these doses, but they are not indications to stop atropine administration. The endpoint of atropinization is drying of respiratory secretions, easing of respiration, and mean arterial pressure greater than 60 mm Hg.13 Animal evidence suggests that early rapid atropinization may limit seizure propagation and, in conjunction with diazepam, prevent status epilepticus.5 Atropine is not active at nicotinic sites and does not reverse the skeletal muscle effects (e.g., muscle fatigue and respiratory failure).2,9 Other anticholinergic medications, such as diphenhydramine or ophthalmic agents, may have benefit if atropine is scarce or unavailable; however, optimal intravenous dosing is not known.14 The second part of acetylcholinesterase inhibition treatment is the use of an oxime, such as pralidoxime (2-PAM, Protopam) or obidoxime (Toxogonin), to regenerate the organophosphate-acetylcholinesterase complex and to restore cholinesterase activity at muscarinic and nicotinic sites.2,4,9,15 There are several dosing regimens; the most common dose of pralidoxime is 1 or 2 g intravenously (pediatric dose, 25-50 mg/kg); additional doses may be given on the basis of clinical response. The medication may be given in a bolus of 1 or 2 g intravenously during 30 to 60 minutes every 4 to 8 hours or 500 mg/hr (pediatric dose, 10-25 mg/kg/hr).15,16 The World Health Organization recommends an initial dose of 30 mg/kg, followed by 8 mg/kg/hr continued for at least 24 hours or, if an infusion cannot be used, 30 mg/kg every 4 hours.17 The infusion may be continued for several days with no adverse effects attributable to the pralidoxime; however, rapid administration can lead to hypertension, vomiting, and a transient reversible neuromuscular blockade.18 The ideal dose of pralidoxime should be determined by monitoring of the clinical condition of the patient and serial cholinesterase levels; the patient may require higher doses of oxime than are recommended here. The World Health Organization–recommended infusion dose of obidoxime is 4 mg/kg, followed by 0.5 mg/kg/hr; alternatively, intermittent intravenous doses of 4 mg/kg, then 2 mg/kg, every 4 hours are given.17 Pralidoxime and obidoxime can be administered by intramuscular injection. Indications for oxime therapy include respiratory depression or apnea, fasciculations, seizures, arrhythmias, cardiovascular instability, and use of large amounts of atropine. Oxime therapy can be used whenever the patient requires more than a limited amount of atropine (2-4 mg) to completely reverse the signs and symptoms of intoxication or in any patient who requires repeated doses of atropine. Oxime therapy and atropine are synergistic. In the past, pralidoxime was used only within the first 24 hours because of aging of the organophosphate-acetylcholinesterase complex, but not all organophosphates behave in a similar manner. Dimethyl and diethyl phosphoryl insecticides react differently at variable rates with acetylcholinesterase and oxime therapy. Many organophosphates are highly lipid soluble and slowly leach out of fat stores for up to 6 weeks, resulting in newly formed complexes with excellent clinical reversal of the cholinesterase inhibition by pralidoxime and by measurements of cholinesterase activity. Pralidoxime can also combine with unbound organophosphates and prevent their subsequent binding to nerve terminals. Even with optimal treatment, seriously intoxicated patients may require long-term supportive care, including ventilator support.4,16 Several studies have looked at the efficacy of pralidoxime.19,20 The results have been mixed and may be due to the variability among different organophosphates. Until this variability is further elucidated, pralidoxime administration in patients with organophosphate toxicity is still recommended. In conjunction with atropine and the oxime pralidoxime, patients with agitation, seizures, and coma should be treated with adequate doses of a benzodiazepine after the airway has been secured.4,5,21 Although diazepam is most studied, any parenteral benzodiazepine may be used. The military has classically used diazepam autoinjectors for intramuscular injection, but midazolam is the best intramuscular agent, with lorazepam as an alternative. Sarin, soman, tabun, and VX are nerve agents that might be used in a terrorist attack. These agents have important differences from the common household or commercial organophosphorus insecticides. These agents tend to age very quickly; tabun (GA) ages in 14 hours, sarin (GB) in 5 hours, soman (GD) in 5 or 6 minutes, and VX in 48 hours. Because of this rapid aging, reversal of nerve agent poisoning with pralidoxime is time sensitive. VX is an oily but highly toxic agent with low volatility. It does not readily vaporize, and because it has a low risk of inhalation, exposure is predominantly transcutaneous. The other agents can be mostly dispersed into the air by explosion or vaporization, resulting in inhalation exposure. These agents do not require the extremely large doses of atropine but do require pralidoxime.22–24 New therapies for treatment of organophosphorus poisoning, including the use of N-acetylcysteine and exogenous acetylcholinesterase, show promise in research studies.25,26 When they are added to anticholinergics, NMDA receptor antagonists may decrease organophosphorus compound–induced seizures.27 Because of the prolonged effects of acetylcholinesterase inhibition, most patients with significant exposures require hospital admission. On occasion, a person with chronic exposure, depressed cholinesterase levels, and mild visual or gastrointestinal symptoms may be observed on an outpatient basis; however, some patients, particularly those exposed to fenthion, initially present with signs and symptoms of mild exposure and progress to severe, life-threatening toxicity over time.28 If plasma cholinesterase levels are available, they may be useful for treatment and disposition decisions. Asymptomatic or minimally symptomatic patients with normal or minimally depressed levels may be discharged after 4 to 6 hours with close outpatient follow-up to ensure that progressive toxicity does not occur. Patients who arrive with known severely depressed levels (usually associated with significant symptoms) require admission and close monitoring, usually in a high-intensity care unit. Patients may have rebound toxicity several days after apparently satisfactory response to initial treatment. Rebound toxicity may occur for many reasons, including persistent release of organophosphates from lipid stores. A secondary syndrome, the intermediate syndrome (IMS), occurs 24 to 96 hours after exposure and consists of proximal muscle weakness specifically of the respiratory muscles. It is believed to be an abnormality at the neuromuscular junction. Patients with IMS present with respiratory failure several days after the acute cholinergic symptoms have resolved and may require several weeks of ventilatory support. It is theorized that this may be a result of inadequate initial oxime treatment or premature discontinuation of oxime therapy.4,29 Oximes may be beneficial for IMS; however, this is controversial.30 Finally, organophosphorus delayed neuropathy has been reported as a different entity. It affects an axonal enzyme, neurotoxic esterase, with a peripheral sensorimotor neuropathy 7 to 21 days after exposure.4 Carbamate insecticides are another class of acetylcholinesterase inhibitors and are differentiated from the organophosphorus compounds by their relatively short duration of toxic effects. Carbamates inhibit acetylcholinesterase for minutes to 48 hours, and the carbamate-cholinesterase binding is reversible. Although the clinical picture of acute carbamate poisoning may be identical to that of organophosphate poisoning, the toxic effects are limited in duration, and patients may require only decontamination, supportive care, and treatment with adequate doses of atropine. Although the duration is limited, patients may become just as ill and require assisted ventilation and seizure therapy. The use of pralidoxime is controversial in carbamate poisoning; an animal study suggests that pralidoxime administration may produce greater toxicity in cases of carbaryl (Sevin) poisoning.31 Nevertheless, if doubt exists as to whether a severe poisoning is due to a carbamate or organophosphate, pralidoxime should be administered. DDT, the prototype of chlorinated hydrocarbon insecticides (sometimes referred to as organochlorine insecticides), was first used extensively during World War II for control of typhus and malaria and widely used in the United States as a general insecticide after the war. Because of the effectiveness of DDT, many other chlorinated hydrocarbon insecticides were developed and used extensively in agricultural, commercial, and residential pest control. Although these insecticides were effective, their widespread use, long half-life, and persistence had negative ecologic repercussions. Many of these insecticides have been targeted as persistent organic pollutants by international agencies, leading to their restricted use.32 Although chlorinated hydrocarbon insecticides are no longer used in the United States for agricultural use, γ-hexachlorobenzene, better known as lindane (Kwell), is still used as a topical medicinal agent for the treatment of head lice and scabies. As a result, lindane is probably the most common cause of toxicity from an organochlorine compound in the United States. Given its toxicity, lindane is no longer a first-line agent for the treatment of scabies.33 In 2001, California issued a ban on the use and sale of lindane, and other states are considering a ban on lindane.32
Pesticides
Organophosphate and Carbamate Insecticides
Principles of Disease
Clinical Features
Salivation
Diarrhea/Diaphoresis
Lacrimation
Urination
Urinary incontinence
Miosis
Defecation
Bradycardia/Bronchorrhea/Bronchospasm
Gastrointestinal cramps
Emesis
Emesis
Lacrimation
Salivation
Complications
Diagnostic Strategies
Management
Disposition
Carbamate Insecticides
Chlorinated Hydrocarbon Insecticides
< div class='tao-gold-member'>
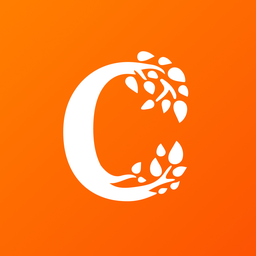
Full access? Get Clinical Tree
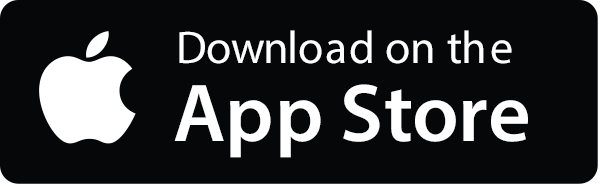
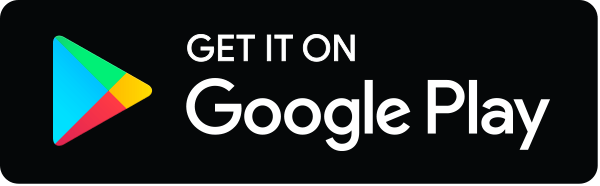