Key Points
- ▪
Intravenous fluid therapy is a core part of perioperative practice, with the potential to influence patient outcomes.
- ▪
Water makes up approximately 60% of total body weight, varying widely with age and body composition. The ratio between the water volume within the intracellular and functional extracellular compartments is approximately 2:1.
- ▪
The endothelial glycocalyx forms a protein-poor intravascular fluid layer at the vessel perimeter; it has been integrated into a revised version of the Starling equation and updated model of capillary fluid movement.
- ▪
Sodium is the dominant extracellular cation and is responsible for much of extracellular fluid osmolality; dysnatremia is typically associated with disturbed extracellular fluid volume.
- ▪
Potassium is the dominant intracellular cation with a key role in the maintenance of transmembrane potentials; dyskalemia may be accompanied by impaired function of excitable tissues.
- ▪
Calcium is a key intracellular second messenger with roles in neuromuscular function, cell division, and oxidative pathways.
- ▪
Magnesium has a diverse range of physiologic effects, underlined by the increasing range of therapeutic applications of supplemental magnesium in the perioperative setting.
- ▪
Acid-base balance is relevant to fluid therapy because fluids containing supraphysiologic levels of chloride may cause an iatrogenic acidosis; the clinical relevance of this acidosis is debated.
- ▪
Intravenous fluids have a range of physiologic effects and should be considered drugs with indications, dose ranges, cautions, and side effects.
- ▪
The physiologic insult of the perioperative period may lead to a wide variety of disturbances in fluid and electrolyte balance.
- ▪
Clinical evidence to guide perioperative fluid therapy is lacking in many areas and cannot be directly extrapolated from general critical care trials.
- ▪
A balance must be found between inadequate fluid administration—allowing tissue hypoperfusion—and the adverse effects of excess intravenous fluids and toxicities related to fluid constituents.
- ▪
Goal-directed fluid therapy (GDT) may help in finding this balance for an individual patient in the perioperative setting, with evidence of reduced postoperative morbidity supporting its use in many surgical settings.
- ▪
No clear consensus exists on which intravenously administered fluid is associated with the best clinical outcomes in the perioperative setting. Comparisons of “balanced” with “unbalanced” and “crystalloid” with “colloid” fluids are being studied in many clinical settings; definitive conclusions are often lacking.
- ▪
The approach to fluid and electrolyte management may need adapting to numerous patient and surgical factors.
The administration of intravenous (IV) fluid is a core expertise for anesthesia providers and an area in which we have an important role in advising clinical colleagues. Alongside the traditional triad of maintenance of unconsciousness, pain relief, and neuromuscular relaxation, IV fluid therapy is a core element of the perioperative practice of anesthesia. The aims of perioperative fluid administration should be to avoid dehydration, maintain an effective circulating volume, and prevent inadequate tissue perfusion during a period when the patient is unable to achieve these goals through normal oral fluid intake.
Knowledge of the clinical effects of different fluids has increased substantially in recent years. The choice of fluid type in a variety of clinical situations can be rationally guided by an understanding of the physicochemical and biologic properties of the various crystalloid and colloid solutions available in combination with the available clinical trial data. Each clinical decision about fluid therapy has two key elements: which fluid to use and how much fluid to give. Recently, several clinical studies have changed our concepts regarding both these questions. However, we should be cautious about overinterpreting data from nonperioperative contexts. Despite recent high-quality “mega-trials” involving thousands of critically ill patients, key questions remain unresolved in the perioperative setting. Goal-directed fluid therapy (GDT) is a good example of an intervention that is effective in the perioperative phase, but ineffective in established critical illness, and this should alert us to other possible similar distinctions. This chapter will review the physiology and pharmacology of IV fluid therapy in humans and discuss the impact of fluid and electrolyte management and alternative approaches on clinical outcomes.
Physiology
Fluid Compartments
Water makes up approximately 60% of total body weight in the average adult, varying with age, gender, and body composition. Adipose tissue contains little water compared with other tissues, leading to marked variability in total body water (TBW) proportion between lean (75%) and obese (45%) individuals. Variation in adipose tissue also contributes to differences in TBW between adult males and females; these differences are reduced in old age as adipose tissue is reduced. The variations in body composition with age lead to a wide variation in TBW ( Table 47.1 ). TBW is divided between anatomic and functional fluid compartments within the body, with the major division between intracellular fluid (ICF) and extracellular fluid (ECF). The size of these compartments and their widely differing composition are shown in Fig. 47.1 and Table 47.2 . The ECF can be subdivided into the following compartments:
- ▪
Interstitial fluid (ISF): Lymphatic fluid and protein-poor fluid occupying cell spaces.
- ▪
Intravascular fluid: Plasma volume, including a proportion contained within the subglycocalyx (see later discussion).
- ▪
Transcellular fluid: Includes gastrointestinal (GI) tract fluid, bile, urine, cerebrospinal fluid, aqueous humor, joint fluid, and pleural, peritoneal, and pericardial fluid. These are functionally important fluids of widely varying composition contained within epithelial-lined spaces and regulated by active cellular transport ( Table 47.3 ).
TABLE 47.3
Composition of Transcellular Fluids (mEq/L Unless Stated)
Data from Grădinaru I, Ghiciuc C-M, Popescu E, et al. Blood plasma and saliva levels of magnesium and other bivalent cations in patients with parotid gland tumors. Magnes Res . 2007;20(4):254–258; Sewón LA, Karjalainen SM, Söderling E, et al. Associations between salivary calcium and oral health. J Clin Periodontol . 1998;25(11 Pt 1):915–919; and Lentner C. Geigy Scientific Tables . Vol. 1. Units of Measurement, Body Fluids, Composition of the Body, Nutrition . 8th ed. Basle: Ciba-Geigy Ltd; 1981.
Daily Volume (L)
Cations
Anions
pH
Fluid
Na +
K +
Ca 2+
Mg 2+
Cl −
HCO 3 −
Gastrointestinal tract
Saliva
1-1.5
30-90
20-40
2.5
0.6
15-35
10-40
6-7
Gastric
1.5-2.5
20-60
10-20
20-160
0
1-3.5
Bile
0.7-1.2
130-150
5-12
10-50
25-100
10-45
7-8
Pancreatic
1-1.5
125-150
5-10
30-110
40-115
8-8.3
Small bowel (concentrations from proximal to distal)
1.8
140-125
5-9
110-60
100-75
7-8
Large bowel
0.2 (lost in feces)
20-40
30-90
0-15
40
7-8
Sweat
0.1-0.5
45-60
5-10
45-60
0
5.2
Cerebrospinal fluid
140
2.8
2.1
1.7
120
7.33
- ▪
Water in bone and dense connective tissue: Constitutes a substantial proportion of TBW but not part of the functional ECF because of slow kinetics of water distribution between this and other compartments.
Age | TBW (%) | ECF (%) | Blood Volume (%) |
---|---|---|---|
Neonate | 80 | 45 | 9 |
6 months | 70 | 35 | |
1 year | 60 | 28 | |
5 years | 65 | 25 | 8 |
Young adult (male) | 60 | 22 | 7 |
Young adult (female) | 50 | 20 | 7 |
Elderly | 50 | 20 |

Extracellular | |||
---|---|---|---|
Intracellular | Intravascular | Interstitial | |
Cations | |||
Na + | 10 | 142 | 145 |
K + | 157 | 4 | 4 |
Ca 2+ | 0.5∗ | 2.5 | 2.5 |
Mg 2+ | 20 | 0.8 | 0.7 |
Anions | |||
Cl − | 10 | 103 | 117 |
HCO 3 − | 7 | 25 | 27 |
HPO 4 2− /H 2 PO 4 − | 11 | 2 | 2 |
SO 4 2− | 1 | 0.5 | 0.5 |
Organic acids | 6 | 6 | |
Protein | 4 | 1.2 | 0.2 |
Total blood volume comprises extracellular (plasma and subglycocalyx compartments) and intracellular (blood cells) elements. With the nonfunctional ECF compartment (bone and connective tissue) excluded, the ratio between ICF and functional ECF is approximately 2:1 (ICF 55% of body weight to ECF 27.5% of body weight).
Physicochemical Laws Governing Fluid and Electrolyte Movement
The movement of water and solutes is governed by a variety of physicochemical and biologic processes, discussed in the following section.
Diffusion
Diffusion is the process by which solute particles fill the available solvent volume by motion from areas of high to low concentration. The speed of this equilibration is proportional to the square of the diffusion distance.
Diffusion also may occur across permeable membranes, according to Fick’s law of diffusion:
J=−DA(ΔcΔx)
Diffusion may also be driven by the tendency of charged solutes to move down electrical gradients.
Osmosis
If a semipermeable membrane (one that is permeable to water but not a solute) separates pure water from water in which solute is dissolved, water molecules will diffuse across the membrane into the region of higher solute concentration. The hydrostatic pressure required to resist the movement of solvent molecules in this way is osmotic pressure. This is one of the fundamental colligative properties of a solution—that is, it depends on the number rather than the type of osmotically active particles in a solution, which may be complete molecules or dissociated ions.
Osmotic pressure in an ideal solution is affected by temperature and volume :
P=nRTV
where P is the osmotic pressure, n is the number of particles, R is the gas constant, T the absolute temperature, and V the volume. The number of particles (n) can be calculated by multiplying (mass of solute/molecular weight of solute) by the number of particles into which the solute dissociates. However, body fluids are not ideal solutions, because interionic interactions reduce the number of particles free to exert an osmotic effect. The total osmotic pressure of plasma is approximately 5545 mm Hg.
Osmolality
Molality is the number of moles (each containing 6 ×10 23 particles of a specific substance ) present in 1 kg of solvent. Osmolality may be used to describe solutions containing many different types of particles and is the number of osmoles (each containing 6 ×10 23 of any type of particle present ) present in 1 kg of solvent. Normal body osmolality is 285 to 290 mOsm/kg and is the same in intracellular and extracellular compartments because of the free movement of water between compartments that consequently prevents the development of any osmotic gradients. The largest contribution to plasma osmolality is made by sodium and its related anions chloride and bicarbonate.
It can be estimated by :
Serumosmolality=(2×Na)+(glucose/18)+(urea/2.8)
Osmolarity is the number of osmoles of solute per liter of solution; unlike osmolality, this may be affected by temperature changes as a result of the volume-expanding effect of increasing temperature.
Tonicity
This is the effective osmolality of a solution with respect to a particular semipermeable membrane and takes into account solutes that do not exert an in vivo osmotic effect. For example, Na + and Cl − do not cross cell membranes freely and therefore exert an effective osmotic force across these membranes, whereas urea freely diffuses across cell membranes and therefore does not exert an osmotic effect here. Similarly, glucose is normally taken into cells by insulin-stimulated facilitated diffusion, so it is an ineffective osmole. Tonicity is important in determining in vivo distribution of fluids across a cell membrane and is sensed by the hypothalamic osmoreceptors. It can be estimated by subtracting urea and glucose concentrations from measured osmolality.
Oncotic Pressure
Oncotic pressure is the component of total osmotic pressure that is due to the colloids, that is, large molecular-weight particles, predominantly proteins (albumin, globulins, fibrinogen). Of the total plasma osmotic pressure of 5545 mm Hg, 25 to 28 mm Hg is due to plasma oncotic pressure. The negative charge on proteins has the net effect of retaining a small excess of Na + ions within the plasma (the Gibbs-Donnan effect), which effectively increases the oncotic pressure above what would be predicted by calculations based purely on protein concentration. As the most abundant plasma protein, albumin is responsible for 65% to 75% of plasma oncotic pressure.
Fluid Compartment Barriers and Distribution
The volume and composition of each fluid compartment depends on the barriers separating it from neighboring compartments.
Cell Membrane
The cell membrane separates the intracellular and extracellular compartments and as a lipid bilayer is impermeable to large hydrophilic molecules and charged particles such as free ions. Other than by passive diffusion of certain molecules, solutes may cross cell membranes in several ways.
Carrier Proteins
Primary Active Transport
Solute transport against a concentration gradient requires energy and is therefore directly coupled to adenosine triphosphate (ATP) hydrolysis—for example, by Na + /K + –adenosine triphosphatases (ATPases). This is the fundamental mechanism by which ionic concentration gradients are maintained, which in turn drive a variety of biologic processes, including water and solute movement and electrical impulse transmission in excitable tissues.
Secondary Active Transport
The process of secondary active transport uses concentration gradients set up by ATPases to transport a solute driven by an ion moving down its concentration gradient, typically Na + . This process is termed cotransport when the solute is also moving down its concentration gradient or countertransport when the solute is being moved against its concentration gradient.
Solute Channels
The solute channels allow much faster transport of solutes than by ATPases or transmembrane diffusion. Examples include voltage-gated Na + channels and the glucose transporter GLUT1, which when inserted into the plasma membrane allows glucose to travel down its concentration gradient. This process is termed facilitated diffusion.
Endocytosis and Exocytosis
The processes of endocytosis and exocytosis are involved in the transport of large proteins and polypeptides across cell membranes.
Vascular Endothelium
The barrier function of the vascular endothelium is particularly relevant perioperatively because of its key role in maintaining intravascular fluid volume. Surgical tissue trauma typically leads to loss of intravascular volume through surgical blood loss or inflammation-related shifts to other tissue compartments. The physiologic effect of IV fluid administered to overcome these losses and maintain adequate tissue oxygen delivery is highly dependent on fluid handling at the capillary level. Our understanding of this area has been refined by experimental physiologic models and techniques.
Capillary Structure
As shown in Table 47.4 , the structure of capillaries varies depending on the underlying organ function. The most common capillary type is the nonfenestrated capillary, comprising continuous basement membrane and a single layer of endothelial cells joined by junctions that are punctuated by breaks. These intercellular clefts are the primary channel for transcapillary fluid flow. The intravascular aspect of the endothelial cells is covered by a continuous network of glycosaminoglycan (GAG) chains, including syndecan-1, hyaluronic acid, and glypican, associated with membrane-bound proteoglycans; and glycoproteins, together forming the endothelial glycocalyx layer (EGL). The EGL covers fenestrations and intercellular clefts and has a thickness of up to 1 μm. In addition to its functions in preventing platelet and leukocyte adhesion, it has emerged as an important semipermeable layer contributing to endothelial barrier function. Water and electrolytes can move freely across the vascular endothelial barrier through the EGL and then intercellular clefts or through fenestrations in the more specialized capillaries. Proteins were previously thought to be excluded from the ISF at the level of endothelial cells; however, it now appears that this occurs at the level of the glycocalyx. The subglycocalyceal layer (SGL) therefore contains protein-poor fluid; slower protein transport into the ISF may occur across the endothelial cells by endocytosis and exocytosis and by transport through a small number of large pores, forming a gradient in protein concentration from SGL to ISF compartments. The volume of the SGL may be as much as 700 to 1000 mL; this volume therefore forms part of the intravascular volume and has an electrolyte composition in equilibrium with the plasma but a much lower protein concentration because of the effective exclusion of larger molecules by the glycocalyx.
Capillary Function
The movement of fluid across the capillary membrane was initially described by Starling and then further refined. A hydrostatic pressure gradient at the arteriolar end of the capillary, greater than the inward oncotic pressure gradient, leads to net filtration of water into the ISF. Much of this water was previously thought to be reabsorbed into the vascular space toward the venular end of the capillary, where the outward hydrostatic pressure is lower and the inward oncotic pressure gradient is increased by exclusion of proteins from the capillary filtrate by the capillary endothelium. The water not reabsorbed by the capillary is removed from the ISF by the lymphatics.
More recent experimental and modeling techniques have integrated the role of the glycocalyx into a revised Starling equation and updated model of capillary fluid movement:
Jv=Kf([Pc−Pi]−σ[πc−πsg])
The key differences and their clinical relevance are as follows :
- ▪
At steady state, continuous capillaries do not exhibit fluid reabsorption toward the venous end of the capillary (the “no-absorption” rule). However, overall measured capillary filtration ( J v ) is much less than predicted by the Starling principle, consistent with the larger colloid oncotic pressure (COP) gradient between SGL and capillary (opposing filtration) than between ISF and capillary. The smaller volume of filtrate is returned to the circulation by lymphatics.
- ▪
Plasma-SGL COP difference, not plasma- ISF COP difference, affects J v . However, the no-absorption rule means that artificially raising COP (e.g., by albumin infusion) may reduce J v but will not lead to reabsorption of fluid from the ISF into the plasma.
- ▪
An exception to the no-absorption rule occurs in acutely subnormal capillary pressures; a transient period of autotransfusion may occur, limited to approximately 500 mL. If subnormal pressures persist beyond this, J v will approach zero, but ongoing reabsorption does not occur. Infusion of colloid in this setting will expand plasma volume, whereas infusion of crystalloid will expand total intravascular volume (plasma and EGL); J v will remain close to zero in both cases until capillary pressure rises to normal or supranormal levels.
- ▪
At supranormal capillary pressures, COP difference is maintained and J v is proportional to the hydrostatic pressure difference. In this setting, colloid infusion will maintain plasma COP but raise capillary pressure further and increase J v . Crystalloid infusion will also increase capillary pressure but reduce plasma COP and therefore increase J v to a greater extent than colloids.
The revised EGL model of fluid distribution, including the proposal that the intravascular volume effects of crystalloids and colloids are partly dependent on the preexisting capillary pressures (context sensitivity), helps explain some of the apparently conflicting findings in clinical fluid research.
Crystalloid Versus Colloid Intravascular Volume Effects
Infused crystalloid has been thought to distribute evenly throughout the extracellular compartments as a result of capillary filtration ( J v ), leaving approximately one fourth or one fifth of the original volume within the circulating blood volume, whereas colloids were presumed to initially remain largely within the intravascular volume. However, many studies of the effects of fluids on blood volume are based on red blood cell (RBC) dilution and changes in the hematocrit and do not account for the influence of the SGL volume, from which RBCs are excluded. Colloids are also excluded from the SGL; by remaining in the plasma volume, they will have a diluting effect on the hematocrit and appear to remain within the circulating volume. Crystalloids initially distribute throughout the plasma and SGL volumes. As a result, their RBC dilutional effects are less than those of colloids. This has previously been interpreted as crystalloid leaving the circulating compartment and entering the ISF; however, a proportion of the infused crystalloid will remain in the blood volume within the SGL. Furthermore, context sensitivity is responsible for the observation that clearance of crystalloid from its central compartment (the intravascular volume) is slower under anesthesia than in awake subjects. It may also explain why the amount of crystalloid required to get intravascular volume effects similar to colloid is in the ratio 1.5:1 rather than the predicted 4:1. The value of this ratio in the perioperative context is less clear and has been inferred from large clinical trials in critically ill patients. However, it is likely to be closer to the measured values in critical illness than the theoretic values traditionally used.
Failure to Reduce Edema By Increasing Capillary Colloid Oncotic Pressure
Hypoalbuminemia is well recognized as a marker of disease severity in critical illness. However, administering exogenous albumin or other colloids to increase capillary COP does not reduce peripheral or pulmonary edema, nor improve overall outcomes in sepsis. The no-absorption rule can provide a partial explanation, because even increasing COP gradient across the capillary wall by administration of albumin will not lead to reabsorption of fluid from edematous tissues. Again, previous studies showing apparent shifts of fluid from the interstitial to the intravascular compartment based on a reduced hematocrit after albumin infusion do not account for the potential role of compaction of the glycocalyx layer and transfer of fluid from the SGL to the plasma volume.
Finally, the importance of the endothelial glycocalyx is highlighted by studies showing that its degradation significantly impairs endothelial barrier function. A range of physiologic insults may lead to glycocalyx injury and shedding, with the subsequent appearance of free heparin, chondroitin, and hyaluronic acid in the plasma. These include natriuretic peptides (which may be released in acute excessive increased intravascular volume), hyperglycemia, and inflammatory mediators released during surgery, trauma, and sepsis, such as C-reactive protein, bradykinin, and tumor necrosis factor (TNF). Glycocalyx degradation may make an important contribution to the already well-characterized endothelial dysfunction seen in inflammation, in which phenotypic changes occur in endothelial cells. Here, an increase in the number of large pores, and a reduction in interstitial hydrostatic pressure favor J v , with an increase in edema in compliant tissues such as the lung, muscles, and loose connective tissue. Impaired glycocalyx function will further favor J v and lead to endothelial platelet aggregation and leukocyte adhesion. Maintenance of glycocalyx integrity is therefore gaining interest as a therapeutic target in perioperative fluid management.
Physiologic Control of Overall Fluid Balance
In health, 60% of daily water loss is through urinary excretion, although this proportion is less when sweating and insensible losses are increased. Integrated cardiovascular and renal neuroendocrine mechanisms attempt to maintain fluid volume homeostasis in response to perioperative challenges, such as reduced oral fluid intake, blood loss, and IV fluid administration.
TBW volume is controlled by a system of sensors, central control, and effectors. The sensors are (1) hypothalamic osmoreceptors that respond to changes in ECF tonicity, (2) low-pressure baroreceptors in the large veins and right atrium that sense central venous pressure (CVP), and (3) high-pressure baroreceptors in the carotid sinus and aortic arch that sense mean arterial pressure. The sensory inputs are integrated within the hypothalamus, which then triggers either increased water intake from thirst or increased water output via antidiuretic hormone (ADH, arginine vasopressin) secretion. Thirst and ADH release may be triggered by increased plasma tonicity, hypovolemia, hypotension, and angiotensin II. ADH release also may be stimulated by stress (including surgery and trauma) and certain drugs (e.g., barbiturates). Water intake does not usually depend on thirst because of social drinking behavior; thirst acts as a backup mechanism when the normal intake is inadequate. ADH, produced in the hypothalamus and released from the posterior pituitary, acts on the principal cells of the renal collecting ducts, which in the absence of ADH are relatively impermeable to water. ADH combines with the vasopressin 2 (V2) receptors on the basolateral membrane of the cells, triggering cyclic adenosine monophosphate (cAMP)-mediated insertion of aquaporin 2 water channels into the apical membrane. This results in water reabsorption down its osmotic gradient and formation of concentrated urine.
Acute Disturbances in Circulating Volume
Acute variation in the intravascular volume leads to compensatory mechanisms over minutes to hours in an attempt to correct the acute abnormality. The homeostatic processes occurring in response to rapid blood loss are aimed at minimizing the change in effective blood volume (venoconstriction and mobilization of venous reservoirs, limited autotransfusion from ISF to plasma, reduced urine production) and maintenance of cardiac output and arterial pressure (tachycardia, increased inotropy, and vasoconstriction). The sensor organs for the acute change are the low-pressure and high-pressure baroreceptors, and initial changes are mediated through increased sympathetic outflow. Renal vasoconstriction leads to a reduced volume of filtrate and activates the renin-angiotensin-aldosterone (RAA) axis. Renin is released from the juxtaglomerular cells and cleaves angiotensinogen to form angiotensin I, which is rapidly converted to angiotensin II. This induces further sympathetic activity, vasoconstriction, aldosterone release from the adrenal cortex, and hypothalamic ADH production. The overall result is increased renal salt and water retention, increased peripheral vascular resistance, and increased cardiac output. In the absence of ongoing loss, the delayed responses to major blood loss restore plasma volume within 12 to 72 hours, increase hepatic plasma protein synthesis, and restore RBC levels by erythropoiesis within 4 to 8 weeks.
Conversely, the rapid infusion of fluid to a normovolemic healthy adult leads to an initial rise in venous and arterial pressure and cardiac output. Several mechanisms act rapidly to bring these cardiovascular parameters toward normal, including pressure receptor-mediated venodilation and venous blood pooling and reduction in systemic vascular resistance. At a tissue level, autoregulatory responses lead to arteriolar vasoconstriction to maintain constant blood flow in the face of increased perfusion pressure. Multiple mechanisms then act to return circulating volume toward normal. A proportion of the infused fluid will be lost as a result of capillary filtration, particularly if the infused fluid reduces COP. Low-pressure baroreceptor stimulation leads to a decrease in pituitary ADH secretion, allowing diuresis, and atrial stretch leads to atrial natriuretic peptide (ANP) release, favoring natriuresis. Further ADH-independent renal mechanisms include glomerulotubular imbalance resulting from the marginal reduction in plasma COP; this rapidly increases the glomerular filtration rate (GFR) and reduces proximal tubule water and Na + reabsorption, increasing urine volume. Finally, increased arterial blood pressure promotes the excretion of excess water and salt (i.e., pressure natriuresis and pressure diuresis). This is the pressure-volume control mechanism, one of the key mechanisms for the long-term maintenance of normal blood volume. However, arterial blood pressure is only slowly restored by cardiovascular reflexes after acute hypervolemia. It may take several days for a 20 mL/kg dose of isotonic salt solution to be fully excreted. Excretion of excess Na + and water depends more on these passive processes and suppression of the RAA axis than on natriuretic peptide activity. The contrast between this inefficiency and the rapid, effective mechanisms for dealing with reduced fluid volume and Na + content reflects the evolution of physiology in an environment with a paucity of salt and variable water availability; excess Na + intake is a feature of modern diets.
Long-Term Control of Circulating Intravascular Volume
The Guyton-Coleman model is the archetypal representation of the circulation. Despite calls to refine the mathematic modeling of the long-term control of arterial blood pressure, it remains the most widely used model to explain the chronic control of blood volume and arterial pressure. In health, short-term variations in blood volume are very small and the cardiovascular system behaves as a closed system with arterial pressure a product of peripheral resistance, vascular compliance, and the Starling curve. In the chronic setting or in acute alterations in blood volume, as described earlier, the circulating volume will vary and equality of input and output must be restored to avoid chronic fluid retention or dehydration; thus, the circulation acts as an open system. The kidneys are the primary organ regulating this equilibrium, largely through pressure natriuresis and diuresis. Indeed, in the chronic setting, arterial pressure subserves the renal requirement to excrete ingested Na + and water rather than simply being a product of cardiac output, vascular compliance, and resistance. A recent interpretation integrates the Guyton-Coleman model with experimental observations ( Fig. 47.2 ). In health, the pressure-natriuresis curve is relatively flat, and excess intake of salt and water can be excreted without long-term rises in circulating volume or blood pressure. In many models of chronic hypertension, the renal excretion mechanism is reset such that natriuresis occurs only at higher arterial pressures and excessive exogenous water and salt results in higher blood pressure.

( Q ˙ )
and peripheral resistance (R) . (B) Experimental models of hypertension (e.g., long-term angiotensin II infusion) with controlled sodium intake (and therefore excretion) demonstrate a reset pressure-natriuresis curve in hypertension. This may be represented by kidney holes positioned further up the arterial column. Natriuresis occurs to a degree similar to that in normotension, so as to maintain a stable body water volume, but requires a higher arterial pressure to do so. P v , Venous pressure.
Electrolyte Physiology
Sodium Physiology
Na + is the dominant extracellular cation, and along with its associated anions accounts for nearly all the osmotically active solute in plasma and interstitial fluid. The relatively free movement of water throughout the fluid compartments means that Na + is therefore the prime determinant of ECF volume. Total body Na + content is approximately 4000 mmol, of which only 10% is intracellular. The concentration gradient between the intracellular and extracellular compartments (ratio 1:15) is maintained by ATPases and is vital for the function of excitable tissues, including action potentials and membrane potential, and for the handling of renal solute.
Na + intake is typically far in excess of minimum daily requirements, which are 2 to 3 mEq/kg/day at birth and decrease to 1 to 1.5 mEq/kg/day in adulthood. Na + is actively absorbed from the small intestine and colon under the influence of aldosterone and the presence of glucose in the gut lumen. Loss is predominantly by the renal route, with minor contributions from feces, sweat, and skin (10 mEq/day each). Na + is freely filtered at the glomerulus, of which 99.5% is reabsorbed, mainly at the proximal convoluted tubule. Serum Na + concentrations are maintained within a tight range (138-142 mEq/L) despite wide variation in water intake by the systems involved in the control of circulating volume outlined previously:
- ▪
hypothalamic osmoreceptor: ADH release
- ▪
atrial volume sensing: ANP release
- ▪
juxtaglomerular apparatus (renal arteriolar baroreceptor and filtrate NaCl content sensing): RAA activation
The excretion of total body excess Na + relies on inefficient passive mechanisms, particularly the pressure-volume effect. Long-term ingestion of excess salt combined with low potassium ingestion contributes to hypertension, a condition not seen in populations with daily salt intake less than 50 mmol. The mechanism involves renal salt retention and initial extracellular volume expansion (later mitigated by pressure natriuresis), with release of an endogenous digitalis-like factor and stimulation of renal Na + pumps, furthering renal Na + retention. Low K + combined with the chronic action of digitalis-like factor inhibits vascular smooth muscle cell Na + /K + ATPases, resulting in excess intracellular Na + content and reduced intracellular K + , smooth muscle contraction, and increased peripheral vascular resistance.
Potassium Physiology
K + is the dominant intracellular cation in the body, with a total body content of approximately 4000 mmol, 98% of which is intracellular, particularly in muscle, liver, and RBCs. The ratio of ICF to ECF K + balance is vital in the maintenance of cellular resting membrane potential, and K + therefore has a key role in the behavior of all excitable tissues. Daily requirements reflect age and growth, with more K + required at higher metabolic rates. Term infants require 2 to 3 mEq/kg/day and adults 1 to 1.5 mEq/kg/day. Nearly all ingested K + is absorbed by the intestine, and minimal amounts are excreted in feces. The acute and chronic handling of K + must therefore maintain a stable plasma K + concentration and resting membrane potential in the face of a daily K + intake of a similar magnitude to the entire ECF K + content. Transmembrane potentials particularly depend on K + permeability, with K + egress occurring through ion channels down its concentration gradient. This leaves behind intracellular anions, with a resultant negative transmembrane potential. The resting value of this potential is achieved when the tendency of K + to move extracellularly as a result of its concentration gradient is matched by the tendency of K + to move intracellularly because of the electrical gradient.
Acute K + distribution involves shifts in K + between the ECF and ICF, performed by ion transport systems under the influence of insulin, catecholamines, and ECF pH. The cell membrane Na + /K + ATPase exports three Na + for every two K + imported and is the means by which the gradients of these ions are maintained. Insulin, released after ingestion of K + -containing food, stimulates the Na + /H + antiporter, increasing intracellular Na + , which is then removed by Na + /K + ATPase with the net cellular uptake of K + . Conversely, in the presence of hypokalemia, skeletal muscle expression of Na + /K + ATPase is reduced, allowing a “leak” of K + from the ICF to ECF. Catecholamines activate β 2 -adrenoceptors, which ultimately stimulate Na + /K + ATPase activity, leading to increases in ICF K + , a mechanism that counteracts the release of K + from muscle cells during exercise. 21 ECF pH also has a bearing on K + handling. The presence of mineral organic acids (where the acid anion is unable to diffuse into the cell) leads to increased cellular H + uptake in exchange for K + ions with consequent increases in ECF K + . Organic acids (e.g., lactic acid and ketone bodies) are more able to diffuse across cellular membranes and H + /K + exchange is much less. Hyperkalemia may be observed in the setting of organic acidemia resulting from alternative mechanisms, such as insulin deficiency and osmotic drag in diabetic ketoacidosis or a failure of ATP production for Na + /K + ATPase in situations typified by anaerobic metabolism and lactic acidosis. Other factors that may influence ECF to ICF K + balance include aldosterone (which at high levels may induce intracellular K + shift, beyond its renal effects), hyperosmolar states (solvent drag of K + along with water efflux), and digoxin (inactivates Na + /K + ATPase and may cause hyperkalemia).
Chronic K + distribution involves renal mechanisms. K + is freely filtered at the glomerulus, and undergoes extensive unregulated reabsorption along the proximal tubule, with only 10% to 15% reaching the distal nephron, where its reabsorption or secretion is tightly controlled. This occurs predominantly in two cell types found in the collecting ducts.
Principal Cells
The principal cells are able to secrete K + under the electrochemical gradients set up by basal Na + /K + ATPases, which maintain low intracellular Na + concentrations (aiding Na + reabsorption from the tubule via Na + channels) and high intracellular K + concentrations (favoring K + secretion into the tubule via K + channels). Principal cell behavior is influenced by the following:
- ▪
Aldosterone, synthesized and released by the adrenal glands in response to raised K + concentrations. This mineralocorticoid increases the synthesis and activity of both basal Na + /K + ATPases and luminal K + channels to drive urinary K + secretion.
- ▪
Tubular Na + delivery. Increased distal tubular Na + content leads to a steeper Na + concentration gradient and increased principal cell reabsorption of Na + . To maintain electroneutrality of the tubular fluid, K + efflux into the tubule increases; this is partly responsible for the hypokalemia associated with diuretics that increase delivery of Na + to the cortical collecting ducts (thiazides and loop diuretics). In contrast, amiloride blocks the principal cell luminal Na + channel and therefore does not affect K + efflux here.
Intercalated Cells
In addition to basal Na + /K + ATPases, these cells have luminal H + /K + ATPases that excrete one hydrogen into the tubule for every K + reabsorbed. Low K + settings lead to up-regulation of this luminal antiporter, reabsorbing more K + at the expense of renal acid loss.
In addition to mechanisms involving aldosterone in a feedback loop, it is likely that feed-forward mechanisms also exist to rapidly modulate renal K + handling when K + is sensed in the GI system, even before plasma K + levels rise.
Calcium Physiology
Beyond its role in bone structure, where 98% of body calcium (Ca 2+) is stored, Ca 2+ is one of the body’s most important intracellular second messengers, playing a key role in muscular contraction, neuromuscular transmission, cell division and movement, and oxidative pathways. Intracellular Ca 2+ entry may have direct effects in cardiac and skeletal muscle contraction—for example, leading to neurotransmitter release or inducing further large-scale release of Ca 2+ from intracellular stores (Ca 2+ -induced Ca 2+ release). A large ECF-to-ICF gradient of ionized Ca 2+ is maintained by ATPases, and cytoplasmic free Ca 2+ levels are kept low by pumping into the sarcoplasmic reticulum. Increases in cytoplasmic Ca 2+ concentration occurring as a result of cellular energetic failure and impaired Ca 2+ transport are a key mediator of cell death pathways. Ca 2+ also plays a key role in coagulation by linking coagulation factors to the negatively charged plasma membrane of activated platelets.
Homeostatic mechanisms maintain serum Ca 2+ concentrations between 4.5 and 5 mEq/L (8.5-10.5 mg/dL), largely under the influence of vitamin D and parathyroid hormone (PTH). Ionized Ca 2+ is sensed by the extracellular domain of a G-coupled receptor expressed on parathyroid cells (the Ca 2+ /Mg 2+ -sensing receptor), inhibiting PTH release. When ionized Ca 2+ levels decrease, PTH is rapidly released with the following actions:
- ▪
Stimulates osteoclast bone resorption, releasing Ca 2+ into the ECF
- ▪
Stimulates distal tubule calcium reabsorption
- ▪
Stimulates the renal conversion of 25-(OH)-vitamin D to 1,25-(OH) 2 -vitamin D (calcitriol, the most active vitamin D metabolite)
The manufacture of active vitamin D involves cholecalciferol formation in the skin during exposure to ultraviolet light, which then undergoes hepatic hydroxylation to 25-hydroxy-calciferol, then renal hydroxylation under the influence of PTH to 1,25-dihydroxycalciferol (calcitriol). As with PTH, this stimulates osteoclastic bone resorption and additionally stimulates absorption of Ca 2+ from the GI tract.
Ca 2+ homeostasis is interlinked with that of other anions. In particular, magnesium is also able to modulate PTH levels, and hypocalcemia and hypomagnesemia frequently coexist. The homeostasis of phosphate (PO 4 3− ) is effectively the converse of Ca 2+ (e.g., renal hydroxylation of vitamin D is inhibited by hyperphosphatemia), and in health the (calcium × phosphate) product is kept relatively stable. An increase in the (calcium × phosphate) product may be seen in advanced chronic kidney disease and is associated with ectopic bone deposition.
Approximately 50% of circulating Ca 2+ is in the biologically active ionized form (the remaining 40% are bound to proteins, predominantly albumin and globulins, and 10% are complexed to anions such as HCO 3 − , citrate, sulfate, PO 4 3− , and lactate). Hypoalbuminemia decreases the total serum Ca 2+ but has less effect on the biologically important ionized form. To calculate the corrected total Ca 2+ concentration, 0.8 mg/dL is added per 1 g/dL decrease in albumin concentration below 4 g/dL. The degree of albumin-protein binding is affected by pH, with acidemia reducing protein binding and increasing the ionized fraction. Ionized Ca 2+ rises by approximately 0.1 mEq/L per 0.1 decrease in pH. Given the approximate nature of corrected total Ca 2+ , biologically active ionized Ca 2+ should be measured when possible. Specimens should ideally be taken without tourniquet (uncuffed), because local acidosis increases the ionized fraction.
Magnesium Physiology
Mg 2+ has a diverse range of cellular actions, including modulation of ion channel activity and as an essential component of ATP production and hydrolysis. It is primarily an intracellular anion, although most is sequestered within organelles, bound to phospholipids, proteins, and nucleic acids. Free ionized Mg 2+ levels within the cytoplasm and ECF are therefore low (0.8-1.2 mM), and chemical concentration gradients are much less than for other anions. Of total body Mg 2+ , 50% is within bone, 20% within muscle, and the rest in liver, heart, and other tissues. Only 1% is within the ECF, and normal plasma levels may be maintained in the face of total body Mg 2+ depletion. Within the plasma, total Mg 2+ concentration is 1.5 to 2.1 mEq/L, of which approximately 25% is protein (mostly albumin) bound, 65% is in the biologically active ionized form, and the remainder is complexed to phosphates, citrates, and other anions. Measurement of ionized Mg 2+ can be performed, although correction is required for interference from Ca 2+ ions. The key roles of Mg 2+ ( Table 47.5 ) highlight its diverse range of clinical applications when administered exogenously and stem from the following three main cellular actions:
- 1.
Energy metabolism: Mg 2+ is required for ATP phosphorylation reactions, interacting with the outer two PO 4 3− groups of ATP. Intracellular Mg 2+ deficiency therefore impairs any enzyme systems using high-energy PO 4 3− bonds, such as glucose metabolism.
- 2.
Nucleotide and protein production: Mg 2+ acts as a cofactor in every step of DNA transcription and replication and messenger RNA (mRNA) translation.
- 3.
Ion transport: by supporting the activity of ion-pumping ATPases, Mg 2+ helps maintain normal transmembrane electrochemical gradients, effectively stabilizing cell membranes and organelles. In addition, effects on ion channels underlie one of the core functions of Mg 2+ , namely physiologic competitive antagonism of Ca 2+ . This is mediated through inhibition of L-type Ca 2+ channels and extracellular local modification of membrane potential, preventing the intracytoplasmic influx of Ca 2+ from both the ECF and intracellular sarcoplasmic reticulum stores. Mg 2+ also effectively antagonizes N -methyl- d -aspartate (NMDA) receptors within the central nervous system, reducing Ca 2+ entry by specific ion channels. These effects result in inhibition of a diverse array of excitable tissue cellular actions, including neurotransmitter release, muscular contraction, cardiac pacemaker and action potential activity, and pain signal transmission.
System | Effect | Mechanism and Clinical Relevance |
---|---|---|
Neurologic | Reduction in pain transmission | NMDA antagonism. Mg 2+ treatment provides effective perioperative analgesia. |
Reduces neuromuscular transmission | Inhibition of neuronal Ca 2+ influx reduces neuromuscular junction ACh release (and motor end-plate sensitivity to ACh). Hypermagnesemia potentiates the effects of neuromuscular blockade. | |
Sympatholysis | Inhibition of neuronal Ca 2+ influx reduces catecholamine release from adrenal medulla and adrenergic nerve endings. Pharmacologic use of Mg 2+ in obtunding pressor response to intubation or during surgery for pheochromocytoma. | |
Anticonvulsant | Mechanism may relate to NMDA antagonism or cerebral arteriolar vasodilation, possible mechanisms for its efficacy in eclampsia, in which vasospasm has been observed. | |
Cortical depression at high levels | ||
Cardiovascular | Vasodilation | Predominantly arteriolar, because of inhibition of Ca 2+ influx-mediated vascular smooth muscle contraction. Mg 2+ administration typically leads to a minor reflex increase in inotropy despite the direct action of Mg 2+ on reducing cardiac contractility. |
Antiarrhythmic effects | Mixed class IV (Ca 2+ channel inhibition) and weak class I (Na + channel inhibition) effects. Increases atrioventricular nodal conduction time and refractory periods, suppresses accessory pathway transmission, and inhibits early and delayed afterdepolarizations. Clinical use is in supraventricular tachycardias, atrial fibrillation rate control and postoperative prophylaxis, and tachyarrhythmias associated with dyskalemia, digoxin, bupivacaine, or amitriptyline. | |
Improved myocardial O 2 supply-to-demand ratio | Coronary vasodilation in combination with reductions in heart rate and contractility; however, no clear evidence of benefit in the setting of acute myocardial infarction. | |
Respiratory | Bronchodilation | Smooth muscle relaxation. Pharmacologic use of Mg 2+ is in acute bronchospasm. |
Renal | Renal vasodilation and diuresis | Ca 2+ antagonism-related smooth muscle relaxation |
Immune | Antiinflammatory | Pharmacologic doses of magnesium sulfate reduce monocyte inflammatory cytokine production. |
Adaptive immunity | Mg 2+ is required as a second messenger during T-lymphocyte activation. | |
Obstetric | Tocolysis | May be due to smooth muscle relaxation |
Mg 2+ is absorbed from the GI tract by a saturable transport system and passive diffusion, in quantities inversely proportional to the amount ingested. Excretion is via the GI tract (∼60% of the ingested amount) and kidneys. Seventy-five percent is freely filtered at the glomerulus, and proximal tubule reabsorption is minimal, with 60% to 70% being reabsorbed at the thick ascending loop of Henle and 10% reabsorbed under regulation in the distal tubule. The regulation of total body Mg 2+ levels by GI uptake and control of renal excretion is not under the control of a well-defined hormonal feedback loop. Although many factors can influence Mg 2+ reabsorption (particularly PTH but also calcitonin, glucagon, acid-base balance, Ca 2+ and K + levels), the main determinant is the plasma Mg 2+ concentration, sensed by Ca 2+ /Mg 2+ -sensor receptors present on the basal aspect of thick ascending loop cells. Other influences may alter the intracellular-extracellular balance of magnesium distribution. Catecholamines, acting by both α- and β-adrenoreceptors, and glucagon lead to extrusion of magnesium from intracellular stores. Although experimental models have shown that adrenergic stimulation may increase serum Mg 2+ concentrations, decreases in serum Mg 2+ concentrations actually occur after stressors such as surgery, trauma, burns, and sepsis. This may be due to a later phase of catecholamine-driven cellular uptake after the initial Mg 2+ efflux.
Phosphate Physiology
PO 4 3− is the most abundant intracellular anion and helps form some of the most important biologic molecules, including ATP, DNA, and RNA, membrane phospholipids, 2,3-diphosphoglycerate (2,3-DPG), and hydroxyapatite in bone. PO 4 3− is therefore required for energy metabolism, cellular signaling through phosphorylation reactions, cellular replication and protein synthesis, membrane integrity, and O 2 delivery. In addition, the PO 4 3− buffer system is one of the key intracellular buffers. Of total body phosphorus, 80% to 90% is stored in bone, with the remainder in the intracellular (soft tissues and erythrocytes) and extracellular fluid compartments. Normal plasma inorganic phosphates are maintained at 3 to 5 mg/dL, and at normal pH, 80% exists in the divalent (HPO 4 2− ) rather than monovalent (H 2 PO 4 − ) form. Plasma phosphates also include lipid phosphates and organic ester phosphates. Most intracellular PO 4 3− is organic.
The typical daily intake of PO 4 3− (∼1 g) outweighs metabolic requirements, yet 70% is absorbed, leading to postprandial increases in serum PO 4 3− levels that are rapidly dealt with by increased renal excretion. GI uptake occurs predominantly by paracellular diffusion and is unregulated unless PO 4 3− ingestion is reduced, when vitamin D and PTH-stimulated active transport intervene. Plasma inorganic PO 4 3− is freely filtered at the glomerulus, 80% of which is reabsorbed in the proximal tubule and a smaller amount in the distal tubule. Proximal tubule reabsorption is via Na + -dependent cotransporters, the expression and activity of which are under the influence of PTH and PO 4 3− intake.
The regulation of normal PO 4 3− levels is mediated primarily by the PTH and vitamin D systems. Low plasma PO 4 3− levels stimulate 1-hydroxylase activity, with the formation of active vitamin D (1,25-dihydroxycalciferol), which increases GI and renal PO 4 3− absorption. Conversely, PTH release (stimulated by reduced plasma Ca 2+ ) reduces renal PO 4 3− reabsorption. PO 4 3− plasma levels also may be reduced in the short term by cellular uptake in response to dopamine and adrenergic activity and alkalosis and intestinal factors (phosphatonins) released in response to increased intestinal luminal PO 4 3− .
Chloride Physiology
As the second most abundant electrolyte in the extracellular compartment, chloride (Cl − ) has a key role in the maintenance of plasma osmolality, preservation of electrical neutrality, and acid-base status (explained by the Stewart model, see later discussion). Normal plasma values are 97 to 107 mEq/L; Cl − is therefore responsible for nearly a third of plasma osmolality and two thirds of plasma negative charge. Most Cl − intake is derived from dietary NaCl, and the GI tract absorbs and secretes large amounts of Cl − , primarily as gastric hydrochloric acid, but also throughout the intestinal lumen. This cellular Cl − secretion leads to paracellular movement of Na + into the lumen, with water moving down its osmotic gradient to form GI secretions. Cl − excretion is primarily renal, largely in the proximal tubule by passive reabsorption or cotransport. More regulated control of Cl − excretion is performed in the intercalated cells of the distal nephron under the influence of plasma acid-base balance by exchange of HCO 3 − for Cl − .
Acid-Base Disturbances and Fluid Therapy
Acid-base balance in general is discussed in Chapter 48; however, the two key areas in which intravascular fluid therapy may affect acid-base balance are iatrogenic acidosis caused by the administration of Cl − -rich fluids and administration of sodium bicarbonate to correct acidosis. In summary, the interpretation of acid-base balance can be viewed in three main ways: by the Henderson-Hasselbach equation, by anion gap, or by Stewart’s strong ion model. The Henderson-Hasselbach equation represents the HCO 3 − buffer system and has plasma HCO 3 − concentration as an independent determinant of plasma pH. The anion gap model is consistent with the Henderson-Hasselbach equation, because it places changes in plasma HCO 3 − at the core of plasma acid-base balance. It represents a simple method for differentiating causes of metabolic acidosis and is defined as the difference between the most abundant measured cation and anion concentrations in the plasma ([Na + ] + [K + ]) − ([Cl − ] + [HCO 3 − ]). The normal anion gap is 4 to 11 mEq/L, and this difference is represented by “unmeasured” anions (PO 4 3− , sulfate, and anionic proteins). In the presence of excess organic acids (e.g., lactic acid or ketoacid), the accumulation of unmeasured anions is accompanied by a reduction in HCO 3 − to buffer the excess H + ions, leading to an increase in the anion gap. In cases in which Cl − is administered, even if HCO 3 − falls, the anion gap will remain normal.
Stewart’s model of acid-base balance has a different approach and proposes that plasma pH is dependent on the following three independent variables:
- 1.
pCO 2 (the plasma CO 2 tension)
- 2.
A tot , the total plasma concentration of all nonvolatile buffers (albumin, globulins, and PO 4 3− )
- 3.
Strong ion difference (SID), the difference between the total charge of plasma-strong cations (Na + , K + , Mg 2+ , and Ca 2+ ) and strong anions (Cl − , lactate, sulfate, and others). In a more simplified approach, apparent SID is defined as ([Na + ] + [K + ]) – ([Cl − ] + [lactate]). Normal plasma SID is approximately 42 mEq/L, and reductions in SID will lead to a fall in plasma pH.
The Stewart model has caused some controversy by representing HCO 3 − as a dependent variable, but it has utility in explaining acid-base disturbances caused by fluid administration.
Hyperchloremic Acidosis
Administration of fluid with Cl − concentration higher than that of plasma will in sufficient quantities (e.g., 30 mL/kg/h of 0.9% saline) cause a metabolic acidosis due to the Cl − content. This hyperchloremic acidosis may be explained by the Henderson-Hasselbach model of acidosis, where saline infusion causes dilution of bicarbonate and a resultant base deficit, or by the Stewart model. Here, the increasing plasma Cl − concentration reduces apparent SID and therefore reduces plasma pH; the SID of completely ionized saline is zero, therefore its infusion progressively dilutes the normal plasma SID. Similar changes are not seen with solutions containing non-Cl − anions which are metabolized after infusion, such as lactated Ringer solution. Although in vitro as an electroneutral solution it also has an SID of zero, following administration, the lactate undergoes metabolism in patients with intact hepatic function, giving it an effective in vivo SID of approximately 29 mEq/L. This is slightly less than the plasma SID and is enough to counteract any alkalosis caused by dilution of A tot .
Saline-induced hyperchloremic acidosis has a variety of potentially deleterious physiological effects. These include renal vasoconstriction, reduced GFR and reduced renin activity in animal models, and reduced renal cortical perfusion in healthy volunteers. Coagulopathy and gastrointestinal dysfunction have also been suggested. However, it is not clear that acidosis purely attributable to iatrogenic hyperchloremia leads to clinically important morbidity. A meta-analysis of studies comparing saline with balanced perioperative fluid regimes confirmed the presence of hyperchloremia and acidosis postoperatively in the saline groups, but typically these biochemical abnormalities were cleared by the first or second postoperative day. There were no overall differences in markers of kidney injury or need for renal replacement therapy, nor in other morbidities such as clinically important coagulopathy or gastrointestinal symptoms. However, the available trials were relatively small, and higher-risk surgical groups (those with pre-existing impairment of acid-base status, emergency and major surgery) were under-represented. Interestingly, in one trial of patients undergoing renal transplant, saline administration was associated with significant hyperkalemia, presumably caused by cellular potassium extrusion due to extracellular acidosis. It is therefore possible that the acidosis observed due to saline administration has a greater clinical effect on a selected high-risk group. Recent large trials in the emergency department and intensive care settings have shown an increase in a composite outcome of death or adverse renal event when patients are given saline rather than balanced crystalloid. The effect seemed to be greatest in medical patients with sepsis. The difference in composite outcomes was small (15.4% in the saline group, 14.3% in the balanced group), but this should be taken in the context of the large number of hospitalized patients that receive intravenous fluids. Although these trials did not relate specifically to the perioperative setting, an increasingly cautious approach to saline administration seems appropriate.
Bicarbonate Administration
The administration of IV NaHCO 3 to treat metabolic acidosis should be reserved for the emergency treatment of select conditions, including severe hyperkalemia and arrhythmias associated with tricyclic antidepressant overdose. In many other situations, clinical benefit is not apparent, a finding that highlights an important pathophysiologic concept. Acidosis in itself may not be physiologically deleterious; indeed, it is a normal event during strenuous exercise, in which it may aid O 2 offloading to tissues. Perhaps acidosis serves as a marker for the severity of underlying disease processes, such as hypoxia, ischemia, or mitochondrial dysfunction, which cause morbidity without adequate correction. HCO 3 − administration also has the following negative effects :
- 1.
Carbon dioxide production. Most of the HCO 3 − administered is converted to CO 2 , with two important consequences. First, excess CO 2 requires excretion by hyperventilation. Converted HCO 3 − of 100 mEq represents an excess of 2.24 L of CO 2 to be exhaled, which may present a significant physiologic challenge to critically ill patients with preexisting ventilatory impairment. Although this is disputed, the excess CO 2 may also diffuse into the intracellular space, aggravating intracellular acidosis.
- 2.
IV HCO 3 − brings a significant Na + content and therefore osmotic load. This may lead to hyperosmolar hypernatremia, ECF expansion, and volume overload.
- 3.
If renal HCO 3 − distribution is impaired, there may be an “overshoot” toward metabolic alkalosis once the underlying disease process causing the initial acidosis is resolved.
In situations in which HCO 3 − administration is required, the total dose required to correct the base deficit can be calculated using the equation:
Dose(mEq)=0.3×weight(kg)×basedeficit(mEq/L)
Although half this dose is usually given, because of the problems outlined above, treatment should stop once the pH rises above 7.2.
Fluid Pharmacology
Given the diverse range of physiologic effects of administered fluids, and the potentially large volumes which can be administered perioperatively, they should be considered as drugs with specific indications, cautions, and side effects. Many of the fluids available currently were developed several decades ago and entered clinical practice without rigorous analysis of their clinical benefits, or knowledge of their effects at an organ or cellular level. Newer colloid solutions have been approved by regulatory authorities and entered widespread clinical usage based on relatively small trials of efficacy. In some cases, safety concerns such as the impact of colloid-related renal dysfunction have only been highlighted by much later adequately powered trials. The composition of available fluids is shown in Table 47.6 , although not all fluids are available in all countries.
Fluid | Sodium | Potassium | Chloride | Calcium | Magnesium | Bicarbonate | Lactate | Acetate | Gluconate | Glucose (g/L) -1 | Other | Osmolarity | Notes | pH (In Vitro) |
---|---|---|---|---|---|---|---|---|---|---|---|---|---|---|
Plasma | 140 | 5 | 100 | 4.4 | 2 | 24 | 1 | — | — | — | — | 285 | SID 42 | 7.4 |
0.9% NaCl | 154 | — | 154 | — | — | — | — | — | — | — | — | 308 | SID 0 | 6.0 |
1.8% NaCl | 308 | — | 308 | — | — | — | — | — | — | — | — | 616 | ||
0.45% NaCl | 77 | — | 77 | — | — | — | — | — | — | — | 154 | |||
5% dextrose | — | — | — | — | — | — | — | — | — | 50 | 252 | 4.5 | ||
5% dextrose/0.45% NaCl | 77 | — | 77 | — | — | — | — | — | — | 50 | 406 | 4.0 | ||
4% dextrose/0.18% NaCl | 33 | — | 33 | — | — | — | — | — | — | 40 | 283 | |||
Lactated Ringer solution (U.S. composition) | 130 | 4 | 109 | 3 | — | — | 28 | — | — | — | 273 | 6.5 | ||
5% dextrose in lactated Ringer solution | 130 | 4 | 109 | 3 | — | — | 28 | — | — | 50 | 525 | 5.0 | ||
Hartmann solution/compound Na + lactate | 131 | 5 | 111 | 4 | — | — | 29 | — | — | — | 275 | In vivo SID 27 | 6.5 | |
Plasma-Lyte 148/Normosol-R | 140 | 5 | 98 | — | 3 | — | — | 27 | 23 | — | — | 294 | 4-6.5 | |
Plasma-Lyte 56 and 5% dextrose/ Normosol M with 5% dextrose | 40 | 13 | 40 | — | 3 | — | — | 16 | — | 50 | 389 / 363 | 3.5-6 | ||
Plasma-Lyte A pH 7.4 | 140 | 5 | 98 | — | 3 | — | — | 27 | 23 | — | NaOH for pH | 294 | 7.4 | |
Sterofundin | 140 | 4 | 127 | 5 | 2 | — | — | 24 | — | — | Maleate 5 | 309 | 5.1-5.9 | |
Plasma-Lyte R | 140 | 10 | 103 | 5 | 3 | — | 8 | 47 | — | — | 312 | |||
Hemosol | 140 | — | 109.5 | 3.5 | 1 | 32 | 3 | — | — | — | In vivo SID 33 | |||
4%-5% albumin | † | — | † | — | — | — | — | — | — | — | Stabilizer: octanoate (caprylate) | † | 7.4 | |
20% albumin | † | — | † | — | — | — | — | — | — | — | Stabilizer: octanoate (caprylate) | † | ||
Plasmanate: Plasma protein fraction (human) 5% | 145 | 0.25 | 100 | — | — | — | — | — | — | — | 88% human albumin, 12% α-/β-globulins | COP 20 mm Hg | 7.4 | |
Gelofusine (4%) | 154 | — | 125 | — | — | — | — | — | — | — | MWw 30 kDa | Succinylated gelatin | ||
Plasmion/Geloplasma (3%) | 150 | 5 | 100 | — | 3 | — | 30 | — | — | — | MWw 30 kDa | Succinylated gelatin | ||
Isoplex (4%) | 145 | 4 | 105 | — | 1.8 | — | 25 | — | — | — | MWw 30 kDa | Succinylated gelatin | ||
Gelaspan (4%) | 151 | 4 | 103 | 2 | 2 | — | — | 24 | — | — | MWw 30 kDa | |||
Haemaccel (polygeline) | 145 | 5.1 | 145 | 12.5 | — | — | — | — | — | — | MWw 35 kDa | |||
Voluven: Waxy maize HES 6% (130/0.4) | 154 | — | 154 | — | — | — | — | — | — | — | 308 | |||
Venofundin: Potato HES 6% (130/0.42) | 154 | — | 154 | — | — | — | — | — | — | — | ||||
Hetastarch: Waxy maize HES 6% (670/0.75) | 154 | — | 154 | — | — | — | — | — | — | — | 309 | 5.5 | ||
Hextend: Waxy maize HES 6% (670/0.75) | 143 | 3 | 124 | 5 | 1 | — | 28 | — | — | — | ||||
Pentaspan: Pentastarch 10% | 154 | — | 154 | — | — | — | — | — | — | — | MWw 264 kDa | 326 | 5.0 | |
Volulyte: Waxy maize HES 6% (130/0.4) | 137 | 4 | 110 | — | 3 | — | — | 34 | — | — | 287 | |||
Plasma volume: Potato HES 6% (130/0.42) | 130 | 5.4 | 112 | 1.8 | 2 | — | — | 27 | — | — | ||||
Tetraspan: Potato HES 6% (130/0.42) | 140 | 4 | 118 | 5 | 2 | — | — | 24 | 5 | — | ||||
10% Dextran 40 | — | — | — | — | — | — | — | — | — | 50 | 255 | 4.0 |
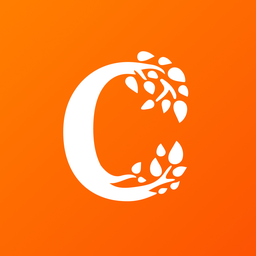
Full access? Get Clinical Tree
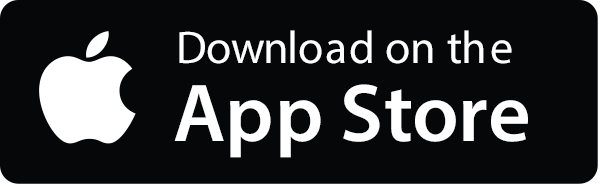
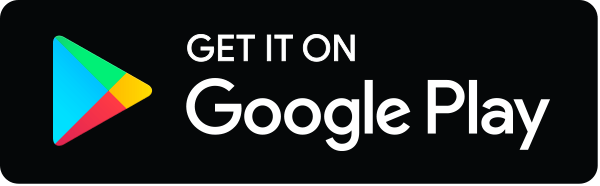
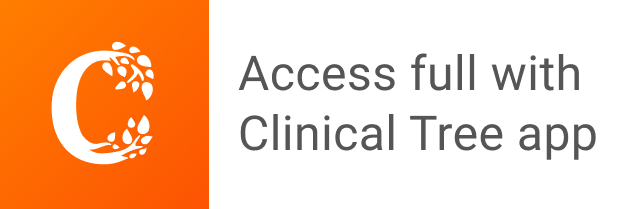