Fig. 17.1
Schematic representation of a cardiopulmonary bypass and ECMO circuit
At the start of surgery, it may be unclear which, if any, mechanical support (CPB versus ECMO) will be required. In the past, we would prepare both ECMO and CPB circuits separately in the operating room (Fig. 17.2a, b). Conversion from ECMO to full CPB may be required in the setting of poor venous return with inability to maintain adequate flows. This could result from blood loss, inadequate drainage from sequestration and cannula position, or kinking. The anesthesia team attempts to compensate for this by administration of volume via their vascular access, but this may not be sufficient or practical. Another reason for conversion to CPB is air entrainment into the ECMO system. During ECMO for LTX, any vascular injury or perforation in close proximity to the drainage cannula can cause air entrainment into the ECMO circuit and air embolism to the patient. Conversion of ECMO to CPB during such critical events can be desperate, clumsy, and potentially hazardous. We developed a hybrid bypass machine that allows a reservoir to be in parallel with, but excluded from, the ECMO circuit to allow conversion to full CPB with only the change of clamp position. This system can easily incorporate a reservoir for rapid fluid administration or removal of large air pockets without the need to change systems or cannula (Fig. 17.3).
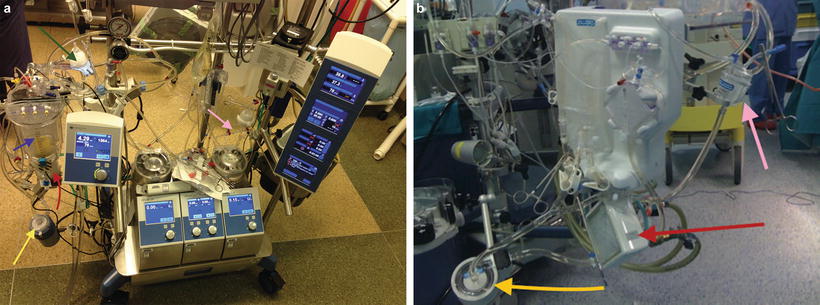
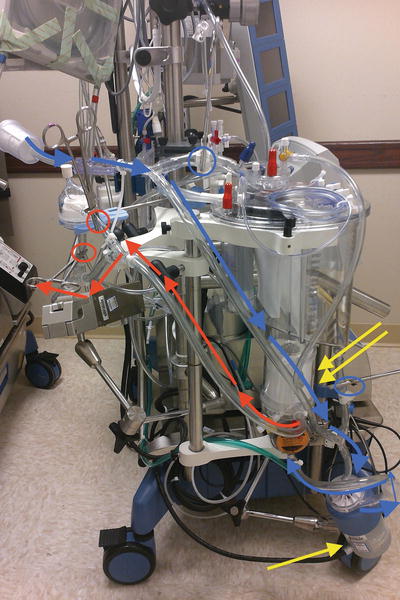
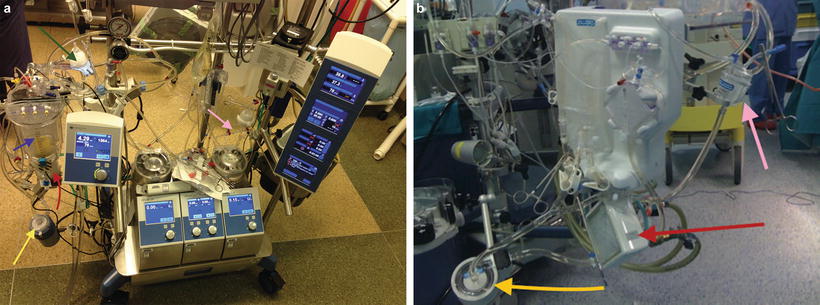
Fig. 17.2
(a) Cardiopulmonary bypass circuit . Blue arrow—venous reservoir, yellow arrow—centrifugal pump, red arrow—oxygenator-heat exchanger, green arrow—arterial line filter, pink arrow—pulmonoplegia delivery assembly. (b) ECMO circuit with oxygenator (red arrow) and pump (yellow arrow)
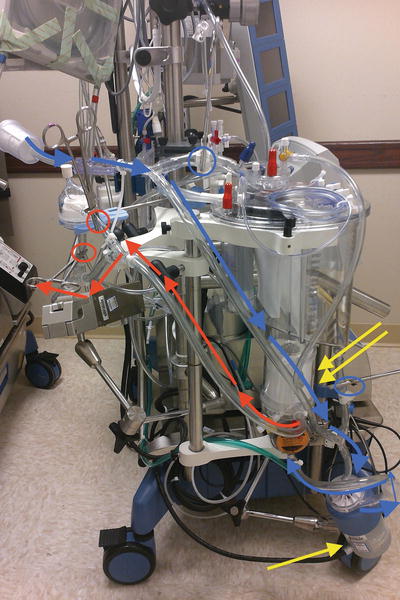
Fig. 17.3
Hybrid cardiopulmonary circuit . Blue and red lines indicate ECMO circuit bypassing venous reservoir (blue circles indicating clamps used to bypass venous reservoir) and arterial filter (red circles indicating clamps used to bypass arterial line filters). Blood flows from venous line, pumped by the centrifugal pump into the membrane oxygenator back into the patient in the ECMO circuit. Clamps shown in figure can be released to convert ECMO circuit to full CPB at any critical period during lung transplantation in this hybrid system. Clamps will be placed on ECMO circuit line before conversion
Cannulation
The cannulation site is variable during transplantation depending on the expected tolerance of anesthetic induction, space available in the chest cavity, peripheral vascular size, and expected difficulty with dissection and duration of expected post-procedure support. Cannulation can be central or peripheral (Fig. 17.4). Central cannulation allows for larger cannula placement and avoids peripheral vascular injury during cut-down, percutaneous access, or dilation. Central cannulation is generally preferred for planned support, given the increased flow capability, but requires dissection and more preparation for direct access, which is not always possible. Central access may be further compromised in the setting of redo surgery when dissection is more difficult and anatomy is less clearly defined.
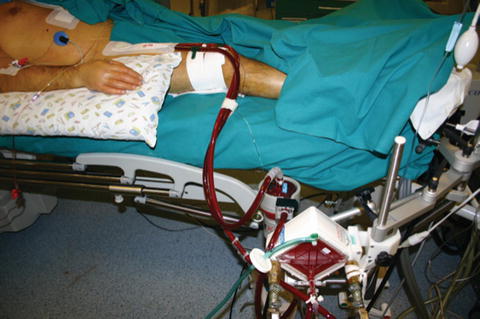
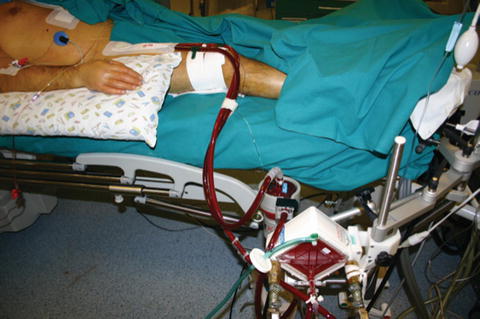
Fig. 17.4
Peripheral femoral venous to femoral arterial ECMO. (© 2013 Formica F, Paolini G. Published in [Formica F, Paolini G. Veno-arterial extracorporeal membrane oxygenation for refractory cardiogenic shock and cardiac arrest. In: Firstenberg MS, editor. Principles and Practice of Cardiothoracic Surgery. Rijeka: InTech; 2013. p. 273–292. DOI: 10.5772/54719] under CC BY 3.0 license)
Venous drainage cannulae are large and range from 10 to 40 French in size. Typically, an average-size patient is drained centrally using a single cannula with openings to receive blood from the IVC and RA (dual stage). Single-stage cannulas drain either the IVC or SVC. Three-stage cannulas have openings to drain the SVC, RA, and IVC through a single cannula.
Peripheral venous drainage of the SVC/RA junction is usually achieved via a long catheter inserted via the right femoral vein. If the patient already has an internal jugular venous cannula for VV ECMO, surgeons will connect the femoral and jugular access using a Y-piece for effective drainage. A peripheral venous cannula can be placed using cut-down or percutaneous methods. We prefer percutaneous access with a modified Seldinger technique of advancement of dilators and cannula over a wire (Fig. 17.5) because of reduced blood loss and infectious complications.
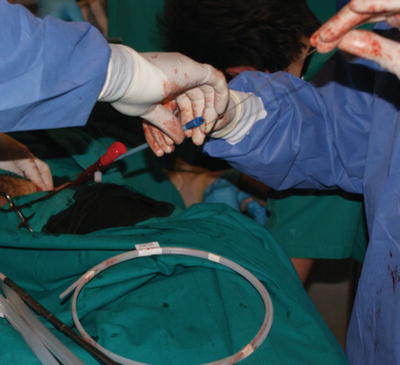
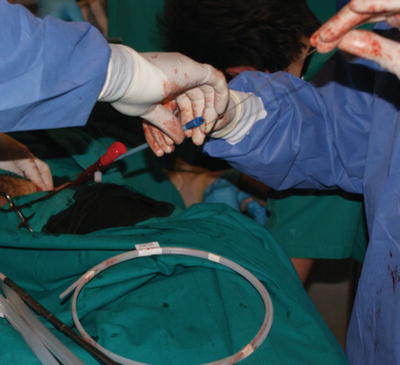
Fig. 17.5
Seldinger technique used for peripheral percutaneous arterial and venous cannulation for ECC. (© 2013 Formica F, Paolini G. Published in [Formica F, Paolini G. Veno-arterial extracorporeal membrane oxygenation for refractory cardiogenic shock and cardiac arrest. In: Firstenberg MS, editor. Principles and Practice of Cardiothoracic Surgery. Rijeka: InTech; 2013. p. 273–292. DOI: 10.5772/54719] under CC BY 3.0 license)
Venous drainage depends on catheter and tubing size, blood volume, height difference between the pump and patient, and the presence of vacuum-assisted drainage (on CPB). Obese patients with higher BSA and flow requirements do not also have concomitant peripheral vascular enlargement to facilitate insertion of bigger size cannula, and thus can be difficult to manage through peripheral cannulation.
Arterial access for CPB and ECMO can be obtained from any major artery, but is generally placed in the ascending aorta (central) or in the femoral artery (peripheral). A variety of cannula shapes and sizes exist. Ideally, flow is directed parallel to the aorta to avoid shear injury to the arterial wall. Cannulation can be direct or wire guided via the Seldinger technique (Fig. 17.5). Just as with venous cannulation, we prefer the percutaneous technique for femoral access. Arterial cannula shapes are variable to facilitate the desired direction of flow. Newer cannulae have thinner walls and multiple openings to improve flow characteristics and reduce vascular shear forces on the aorta. If flow is misdirected up a major arch vessel, hyperperfusion syndrome and a higher embolic burden to that vessel will be the end result. For this reason, we use transesophageal echocardiography (TEE) guidance to assure proper placement (Fig. 17.6a, b). With peripheral cannulation, it should be noted that aortic plaques may be more easily dislodged when aortic flow is reversed. Occlusion of arterial flow because of the catheter can cause distal limb ischemia. In our institution, we place a distal perfusion cannula (5–8 F) that diverts flow to the affected limb (Fig. 17.7) if ischemia develops by physical exam or NIRS desaturation of a limb. Some institutions favor femoral or axillary arterial end-to-side anastomosis with a graft material before cannulating the graft. This is more invasive but precludes limb ischemia because the cannula is not obstructive.
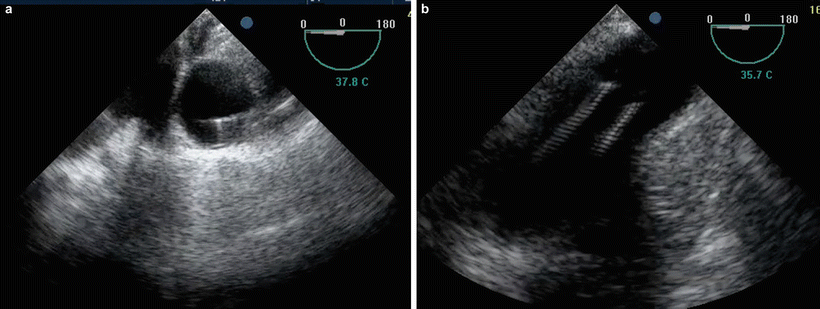
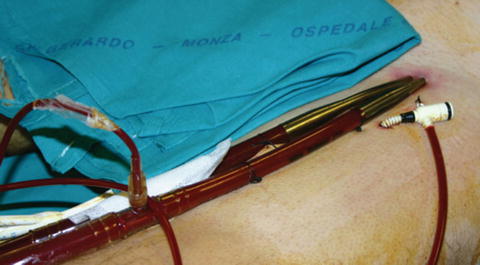
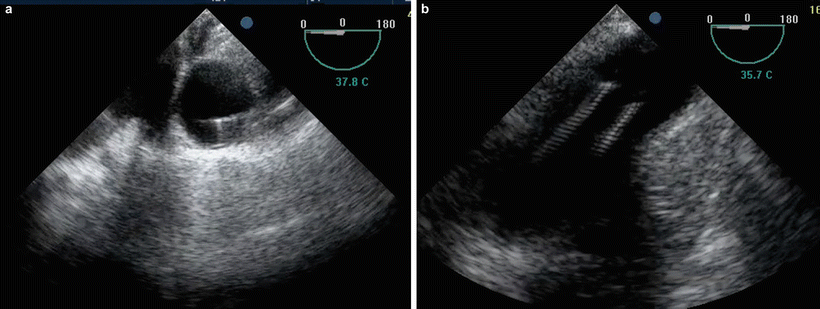
Fig. 17.6
(a) Wire confirmed in the descending thoracic aorta during femoral arterial cannulation (peripheral cannulation). (b) Central aortic cannulation confirmed by the position of the tip of the cannulae in the proximal arch of aorta
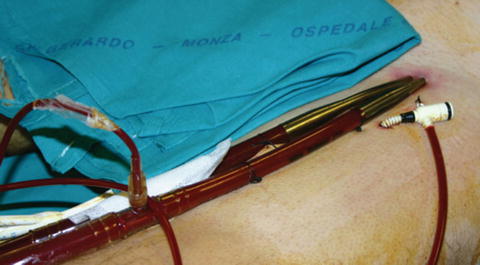
Fig. 17.7
Distal perfusion cannula to decrease limb ischemia during peripheral ECC. (© 2013 Formica F, Paolini G. Published in [Formica F, Paolini G. Veno-arterial extracorporeal membrane oxygenation for refractory cardiogenic shock and cardiac arrest. In: Firstenberg MS, editor. Principles and Practice of Cardiothoracic Surgery. Rijeka: InTech; 2013. p. 273–292. DOI: 10.5772/54719] under CC BY 3.0 license)
Cannula placement in the operating room is confirmed in real time using TEE. TEE-guided procedures add real-time identification of wires prior to vascular dilation and allow accurate cannula positioning without the need for repeated manipulation. Dissection or other vascular injuries are dreaded complications of vascular access and can be monitored with TEE during and after cannulation.
Although pressure and flow are generated by the pump as described below, the resistance to venous drainage and to arterial flow is primarily determined by cannula and tubing diameter. The flow characteristics for each cannula are described in the manufacturer’s documentation, but often underperform the listed benchmark values. The undersizing of cannula can significantly reduce the capability of ECC. Oversized cannulae can cause vascular injury or obstruction. When a cannula occupies much or all of a venous structure, blood flow peripherally will be compromised by venous back pressure. This can result in SVC syndrome or cerebral ischemia. With IVC obstruction, there can be hepatic, renal, bowel, or limb congestion.
Tubing
Clear polyvinyl chloride (PVC) tubing bonded with heparin and other advanced biocompatible coatings connect the components of the bypass circuit together. Enhanced biocompatible coatings reduce surface contact activation, and inflammatory response, and provide improved outcomes such as decreased time to extubation [4]. These tubes can be of any length and diameter, but most institutions use 3/8″ or 1/2″ diameter tubing for venous drainage during CPB and 3/8″ tubing for ECMO. The smaller 3/8″ tubing reduces priming volume and its dilution effect and has been shown to decrease transfusion and the inflammatory response [5, 6].
Use of smaller tubing can result in incomplete drainage of the surgical field and also necessitates the use of a vacuum to achieve sufficient venous drainage. Our microcircuit uses a 3/8″ venous line, a raised reservoir, vacuum-assisted venous drainage (VAVD), and an integrated arterial filter to reduce dilution of the patient. The ½″ pump uses a ½″ venous line with a reservoir placed below the patient’s level to use gravitational forces to assist drainage. The venous line must be fluid filled or an airlock can occur.
During VAVD, negative pressure (maximum −40 mmHg) is applied to the venous reservoir to facilitate venous drainage. This allows initiation of CPB with a dry venous line to prevent further dilution. This form of augmented flow does not come without risks. VAVD can also result in air pulling out of the solution, causing the potential for gaseous microemboli in the patient if the negative pressure is too low. Safety devices on the vacuum regulator include a negativity safety vent that limits suction to −100 mmHg, as well as an excess positive pressure relief valve if the regulator gets over-pressurized. The pump also employs its own pressure relief valve. Air entrainment can create an airlock, stopping effective circulatory support. While an airlock can be remedied mechanically, air embolus can be devastating and has resulted in morbidity as well as frank brain death [7]. The use of assisted drainage also increases hemolysis [8]. Drainage in ECMO is not passive. ECMO is a closed circuit and blood is actively pulled into the system by the negative pressure generated by a centrifugal head (preload dependent).
Improper or loose connections between segments of tubing are a source of airlock and embolism and are therefore securely fastened with locking ties. Tubing may be misconnected at any point. This is more commonly done on the surgical field by misconnecting the venous cannula to the ECC-pressurized outflow and the aortic cannula to the venous drainage. Misconnections can be prevented on many levels. Clear plastic tubing is lined with color coding: blue, yellow, and red. In addition, there is usually a reduced diameter for arterial tubing since the venous outflow is facilitated by larger tubing. Pressure monitoring lines on the arterial circuit should confirm pulsatile pressure that correlates closely with the patient’s arterial pressure prior to initiation of cardiopulmonary support. With initiation of ECC, the arterial pulse pressure should narrow and central venous and PA pressures should fall to zero.
Reservoir
The venous reservoir holds excess volume from the patient that was in the heart and lungs. Reservoirs are present only for true CPB and require intense anticoagulation since blood stagnates in this container. They are usually 3–4 L in capacity. This compartment provides a more consistent source of blood for the pump and eliminates the need for intravascular administration of volume while on bypass. Volume changes in the reservoir can be due to bleeding in the surgical field, surgical manipulations of the heart and major vessels, vascular tone (constriction or dilation), as well as urine output.
There are two types of reservoirs: soft and rigid. We use exclusively rigid reservoirs made of polycarbonate. The benefits of rigid reservoirs are twofold: more accurate volume measurement and automatic air venting. This helps with the estimation of the need for additional volume and estimation of the time before reservoir exhaustion. All CPB systems have level detectors that trigger an alarm and shut off the pump if the volume gets too low to prevent air entrainment into the arterial side of the pump. Rigid containers are automatically vented of air and allow mixing of cardiotomy drains, vents, and cannula drainage. The air-blood interface is a significant source of inflammatory response. A 47 μm screen filter is used to filter the returning venous blood. The screen filter also includes a polyurethane defoamer. The suction side of the reservoir has a depth filter to filter clot and any particulates that may enter the system from the vents and suction from the surgical field.
Soft reservoirs are basically a plastic bag that expands with venous return. As the bag collapses, volume measurements become inaccurate. Air entrained into these containers must be manually aspirated. Additionally, as the soft reservoir fills with fluid or air, it can create back pressure, reducing venous drainage. The benefit of a soft reservoir is a smaller priming volume and less dilution.
Pump
There are two basic pump mechanisms: centrifugal impellers and roller pumps. At our institution, we use exclusively centrifugal impellers for both CPB and ECMO. These pumps generate forward flow through the oxygenators and filters and provide perfusion pressure.
Roller mechanisms are mechanically simple. A roller head in contact with the tubing partially compresses and rolls up the tubing some length before losing contact and another roller head a distance back repeats this action. This forces the blood ahead of the roller forward and creates a vacuum behind, drawing the venous return forward. Each pass of the roller creates a stroke volume and total flow is the product of this volume times the rotations per minute (RPMs). The rollers are set to be partially non-occlusive so as to reduce hemolysis. The amount of occlusion is set by the perfusionist. Formed elements are damaged by both under- and over-occlusion. Over-occlusion causes crush injury to the elements of blood. Under-occlusion results in a high-velocity backflow that causes shear injury to blood. This propulsion is afterload independent. Any partial occlusion, clamping, or kinking distal to the roller pump can cause the tubings to over-pressurize if the safety mechanisms are not activated. This over-pressurization can cause connectors to decouple, as well as cause cracks in the venous reservoir. Safety device alarms will make the perfusionist aware of a high pressure (usually 325–350 mmHg) and the pump will shut off if the pressure reaches a certain level (>375 mmHg). Safety systems will disable pumping when high pressures are sensed, but this reactionary mechanism may not prevent damage to components. On the other hand, an occluded venous return line may result in a cavitation phenomenon behind the roller. In this setting, without additional preload to fill the evacuated stroke volume, negative pressure is created and vaporizes soluble gases into bubbles, which can then be delivered to the patient.
The centrifugal pump head consists of a cone-shaped plastic housing that contains a magnetic impeller. The pump head is seated into the drive console that has magnetic bearings that spin, causing the impeller inside the cone housing to spin. This creates a vortex that pulls blood in by generating negative pressure at the inlet; then, the rotational force of the vortex pushes blood outward, creating positive pressure towards the outlet of the cone housing driving blood to the oxygenator and patient. If the pump becomes entrained with small amounts of air, bubbles will remain in the center of the vortex while denser elements move outward. Massive air intake will disrupt vortex formation and stop pump flow rather than forcing large volumes into the patient.
This centrifugal propulsion differs fundamentally in its reaction to loading conditions and in terms of cellular trauma. The centrifugal pumps are preload and afterload dependent. The pump will not flow unless there is enough negative pressure to pull blood into the system. If the negative pressure is too low, cavitation of the cannula on the patient’s vessels can occur. This can be due to cannula size and/or the volume status of the patient. If there is excessive negative pressure, the inflow line occludes and the pump lowers flows or stops flowing. Excessive negative pressure will also cause hemolysis. Afterload dependence can be seen when flow rate increases without a change in revolutions per minute (RPM), because of a decrease in the patient’s systemic vascular resistance. Increased resistance to pump outflow causes flows to decrease without a change in RPM.
Both pump systems require electricity and most devices have built-in battery backup. Hand cranking of either mechanism is possible if battery reserves should fail during bypass. Modern equipment of either type is highly reliable.
Both roller and centrifugal pumps deliver essentially laminar, nonpulsatile flow. The argument that pulsatile flow (cyclic sheer stress) improves microvascular perfusion is yet unproven. Bench devices and ventricular assist technology will continue to investigate the benefits of pulsatile flow. Currently, the only way to provide this kind of flow intraoperatively is with the addition of balloon counter-pulsation.
Oxygenator/Heat Exchanger
Blood enters the heat exchanger before it enters the oxygenator. The heating and cooling of the patient change the solubility of gases; therefore, heat transfer occurs before oxygenation. Usually heat exchangers are made of stainless steel, aluminum, or polypropylenes, which all have good thermal conductivity. The heat exchanger has a water and a blood side. The blood side has surface agents to minimize blood activation and to maximize heating and cooling. The blood and water pathways flow in a countercurrent direction, which also reduces outgassing of solutions due to rapid changes in temperature. The temperature gradient should be kept at a maximum of 10° between the water temperature and the patient’s blood temperature. Rapid cooling is much better tolerated than rapid rewarming, which can result in vaporization of dissolved gasses and increase the microembolic load.
Blood flows to the oxygenator before being returned to the patient. Historically, bubble oxygenation preceded the use of membrane oxygenators. These have been entirely replaced given the much higher risk of gas embolization that occurs when bubbles are added intentionally to the system. Membrane oxygenators are microporous hollow fiber membranes that have a semipermeable barrier, which separates fluid from the gas phase. The diffusive properties of the oxygenator membrane allow the transfer of O2 and CO2 between the phases by relying on differences in partial pressure of medical gases. Oxygenators work by blending compressed air and O2 to maximize the driving pressure difference for O2 diffusion. The rate of fresh gas flow delivered is called the sweep rate and determines the amount of CO2 removal.
After oxygenation and heating, blood is passed through a filter before being returned to the patient. Filters can be quickly changed if they become saturated with clot. It is important to monitor these filters, since they can be an early warning of ineffective anticoagulation.
Additional Safety Features
A number of other safety devices are installed on the ECC. These include pressure monitoring lines, bubble detection, reservoir exhaustion detection, emergency shutoff mechanisms, and a number of one-way valves to prevent flow reversal. These devices improve the margin of safety in operating these systems.
Much like in anesthesia care, despite all of the technology improvements and monitoring, it is inevitably the human that has the greatest impact on safe operation. The importance of communication and coordination of bypass operations cannot be understated. Checklists improve the reliability of assembly and operation of these complex machines. Transplantation is a team sport; good closed-loop communication, mutual respect, and vigilance are required by all players for optimal performance. All parties have a duty to report concerning or unusual findings or laboratory or monitoring values in real time.
In addition to those functions noted previously, ECCs have a variety of other built-in functions. There are ports for drug administration and blood gas sampling. A typical anesthesia vaporizer allows administration of volatile anesthetic when the lungs are bypassed. Small tubing lines allow recirculation to reduce stagnation and clotting. Additional devices called vents and pump suckers, which recover additional blood, may be incorporated.
“Pump Suckers”/Vents
Surgical dissection can cause significant blood loss depending on the degree of adhesions, coagulation status, and prior surgery. During CPB, blood recovered from the surgical field can be returned to the venous reservoir via pump suckers. LV distension during surgery can be caused by aortic insufficiency, Thebesian drainage, and bronchial drainage. Normally 1 % of cardiac output is directed towards the bronchial vessels. In the setting of advanced pulmonary disease (bronchiectasis), this may increase to as high as 9.3 % [9]. LV distention from these sources increases the oxygen demand of the myocardium. LV vents are used to decompress LV and vents return blood to the circuit. When ECMO is planned, the use of vents and pump suction is not possible. Blood from the field can be recovered through cell salvage, albeit delayed. The advantage of CPB is such that return is immediate and also coagulation factors are not washed from the blood. This blood that has been extracted from the field does appear to have a higher inflammatory cytokine content, which can contribute to systemic inflammation and injury [10]. Aspiration of air, pericardial fat, and non-blood elements through vents and pump suckers may also cause hemolysis or microembolism and initiate the systemic inflammatory response [10].
Modified Ultrafiltration (MUF)/Hemoconcentration
Occasionally, patients present with or develop volume overload, especially in the setting of renal failure. By diverting a portion of the systemic outflow or returning venous blood to a specialized network of hollow fibers with micro-porous membranes, similar in concept to the CPB oxygenator, convective forces extrude plasma volume. These units can effectively remove volume without disrupting the balance of electrolytes or injury to the formed units of blood. They may also be used to minimize the effect of dilution associated with priming before separation from bypass.
Priming
The pump is primed with 1–1.5 L of isotonic fluid. Our institution uses a combination of Plasmalyte A®, heparin, and mannitol. This prime volume can also be used with blood if it is expected that the dilution will lead to unacceptable hemoglobin levels. Retrograde autologous priming (RAP) may be helpful with planned CPB. The primary goal of priming is to remove air bubbles from the circuit, which can be facilitated by gaseous CO2 flush prior to liquid priming. Residual CO2 bubbles are soluble and less harmful if embolized; these are cleared by the oxygenator sweeps during initial circulation testing. Fluid priming allows the pump function to be tested before it is connected to the patient. Following priming, flow is circulated and the circuit is pressurized to ensure ECC integrity. This allows testing of seals, one-way valves, and the mechanical drive system. After successful priming, the sterile portions of the tubing are handed off to the surgical field, where they are cut to length and eventually attached to the cannula.
Cardioplegia
Cardioplegia is delivered with the intent to arrest the heart. This reduces metabolic demands and improves the surgical field of view. Cardioplegia is not used for LTX unless a concomitant intracardiac procedure is performed (aortic valve replacement, mitral valve repair). Antegrade cardioplegia is delivered to the aortic root proximal to the aortic clamp. This closes the aortic valve and pressurizes the root, forcing perfusion of the coronary arteries. Retrograde cardioplegia by way of a coronary sinus catheter placed through the RA is generally avoided during transplantation.
Organ Preservation
Organ procurement and transplantation come with obligatory periods of donor organ ischemia. Efforts to minimize organ damage start prior to procurement with good intensive care unit (ICU) care, minimizing insults and protective ventilation. Following donor death and organ procurement exists an obligatory period of warm ischemia. Traditionally, cold perfusate and packing in cold preservative and ice are used to preserve organs awaiting transplantation. Donor organ temperature is dropped to 4 °C with a 12-fold reduction in metabolism [11]. However, anaerobic metabolism persists with the need for additional protection measures to avoid tissue damage from free radicals, complement activation, leukocyte activation, endothelial injury, cytokine release, and calcium overload [12]. Various perfusion solutions are used along with hypothermia to provide additional organ protection. University of Wisconsin (UW) solution, an intracellular solution used for preservation of the heart, and Perfadex (PER), an extracellular solution, are used for lung protection at our institution (Table 17.1). UW solution has shown a slight survival advantage in heart transplantations, while PER has been shown to improve PaO2/FIO2 ratio and shorten the duration of ventilation for LTX patients [13].
Table 17.1
Preservation solutions during transport of organs for thoracic organ transplantation
UW solution | PER solution | |
---|---|---|
Intracellular/extracellular | Intracellular | Extracellular |
Na+ (mEq) | 25 | 138 |
K+ (mEq) | 120 | 6 |
Impermeant/colloid | LactoB, raffinose, hydroxyethyl starch | Dextran |
Buffer | Phosphate | Phosphate |
Antioxidant | Allopurinol, glutathione | |
Osmolarity (mOsmol/L) | 330 | 292 |
Magnesium | 5 | 0.8 |
Chloride (mEq)
![]() Full access? Get Clinical Tree![]() ![]() ![]() |