Fig. 7.1
Derivation of ester- and amide-class local anesthetics from cocaine. Shown are chemical structures and years in which each drug was first used
7.2.1 Onset of Action, Potency, and Duration
Being weak bases, local anesthetics exist in solution as both ionized (water soluble) and non-ionized (lipid soluble) molecules. Local anesthetics traverse phospholipid membranes in their non-ionized form. The degree of drug ionization is determined by the dissociation constant (pKa) and the pH of the surrounding fluid. The pKa of a molecule represents the pH at which 50 % of the molecules exist in a lipid-soluble form and 50 % in a water-soluble form. Local anesthetic molecules with a pKa that approaches physiologic pH have a higher concentration of the non-ionized lipid-soluble form. As the pKa of a drug increases, a greater proportion exists in the ionized hydrophilic form at physiological pH. Commonly used local anesthetics have a pKa between 7.8 (lidocaine) and 8.1 (ropivacaine and bupivacaine) (Table 7.1). Drugs with a lower pKa (e.g., lidocaine) exist to a greater degree in a non-ionized form and diffuse more easily across cell membranes. This explains why lidocaine has a faster onset of action than ropivacaine or bupivacaine. Moderately lipophilic drugs are most effective clinically due to the drug having to cross several membranes to reach its target site. At physiological pH, a significant fraction of the drug is in a non-ionized form and readily crosses the membrane to the cytosolic side of the nerve cell. Excessively lipophilic drugs remain in the first membrane encountered [6]. For the drug to effectively block the sodium channel, it must become re-ionized on the cytosolic side of the membrane.
Table 7.1
Comparative physicochemical properties of local anesthetics
Agent | Potency | pKa | Molecular weight (Da) | Toxic plasma concentration (μg/mL) | % protein binding |
---|---|---|---|---|---|
Lidocaine | 1 | 7.8 | 234 | >5 | 70 |
Prilocaine | 1 | 7.8 | 220 | >5 | 55 |
Mepivacaine | 1 | 7.6 | 246 | >5 | 77 |
Bupivacaine | 4 | 8.1 | 288 | >3 | 95 |
Levobupivacaine | 4 | 8.1 | 288 | >97 | |
Ropivacaine | 4 | 8.1 | 274 | >4 | 94 |
Agent | % ionized at pH 7.4 | Partition coefficient with h-octanol/buffer | Volume of distribution (L) | Clearance (L/min) |
---|---|---|---|---|
Lidocaine | 25 | 43 | 91 | 0.95 |
Prilocaine | 24 | 25 | 191 | |
Mepivacaine | 39 | 21 | 84 | 9.78 |
Bupivacaine | 17 | 346 | 73 | 0.47 |
Levobupivacaine | 17 | 346 | 55 | |
Ropivacaine | 17 | 115 | 59 | 0.44 |
Potency is directly related to lipid solubility which is expressed as lipid/water partition coefficient. Drugs with low lipid solubility need higher concentrations to produce a block of similar intensity to that produced by local anesthetics with higher lipid solubility (e.g., 2 % lidocaine (partition coefficient 43) vs. 0.5 % bupivacaine (partition coefficient 346)). Duration of action is largely determined by the degree of plasma protein binding.
7.2.2 Sodium Channel
Voltage-dependent Na+ channels in nerves contribute to the control of membrane excitability and are responsible for action potential generation. When the cell membrane is depolarized by a few millivolts, sodium channels activate and inactivate within milliseconds (Fig. 7.2). Influx of sodium ions through the channel depolarizes the membrane further and initiates the action potential. Local anesthetics prevent neural excitation and subsequent propagation of action potential by inhibiting passage of Na+ ions through these channels. The drug may access the Na+ channel through the hydrophilic inner pore or traverse the hydrophobic cell membrane when the channel is closed [7]. The local anesthetic must be re-ionized to prevent passage of Na+ ions. An equilibrium exists between the ionized and unionized forms in the Na+ channel.


Fig. 7.2
Schematic diagram of a sodium channel in a neuron cell membrane. Left, the deactivated form is impermeable to sodium ions; middle, the activated form allows sodium ions to flow into the cell; right, the channel is blocked by local anesthetic. Local anesthetics traverse the phospholipid membrane in their non-ionized form. Drugs with a low pKa (e.g., lidocaine) exist to a greater degree in a non-ionized form and more readily cross the cell membrane. The drug must become re-ionized in the intracellular environment to effectively block the sodium channel
The voltage-gated sodium channel is a large, multimeric complex comprised of an α subunit and one or more smaller β subunits. The α subunit contains all the features necessary for a functional ion channel including voltage sensor, activation/inactivation gates, and ion pore. The β subunits play a role in membrane localization of the channel as well as modulation of channel gating [8]. Na+ channels exist in a closed, open, and inactivated state [7]. Local anesthetics bind more tightly to the Na+ channel during the open and inactivated state than the closed state. In effect, this means that they show high affinity for their site of action during high-frequency action potentials, such as those that occur during sensory transmission or motor activity [9]. This is a phenomenon known as frequency-dependent or use-dependent blockade.
7.2.3 Physiological Considerations
The diameter and degree of myelination of a nerve fiber determines the nature of impulse conduction and the effectiveness of local anesthetic drugs. There are three major anatomical categories: myelinated somatic nerves (A fibers), myelinated preganglionic autonomic nerves (B fibers), and unmyelinated axons (C fibers). The A fibers are subdivided into four groups according to decreasing speed of impulse conduction and diameter (Table 7.2). The thinnest A fibers, the Aδ group, are responsible for pain and cold temperature transmission. Aγ fibers are responsible for muscle tone. C fibers are thinner than myelinated axons, have a much lower conduction velocity, and are also responsible for the transmission of pain signals. Aδ fibers are responsible for fast pain transmission, whereas C fibers are responsible for second or slow pain transmission [6]. Differential nerve blockade has been demonstrated in animal studies after administration of local anesthetic (i.e., fibers of smaller diameter are blocked before those of larger size with recovery from blockade occurring in the reverse order) [10]. Clinically, it is more likely that the action of local anesthetic on nerve fibers is multifactorial (e.g., peripheral versus core nerve fiber, state of activation of the nerve, length of nerve exposed to local anesthetic, and degree of myelination in addition to nerve diameter).
Table 7.2
Classification and properties of peripheral nerve fibers
Nerve class | Aα | Aβ | Aγ | Aδ | B | C |
---|---|---|---|---|---|---|
Function | Motor | Pressure/touch | Proprioception/motor tone | Pain/temperature | Preganglionic autonomic | Pain/temperature |
Diameter (μm) | 12–20 | 5–12 | 1–4 | 1–4 | 1–3 | 0.5–1 |
Conduction speed (m/s) | 70–120 | 30–70 | 10–30 | 12–30 | 10–15 | 0.5–1.2 |
Local anesthetic sensitivity (+++ most susceptible) | ++ | ++ | +++ | +++ | +++ | + |
Myelination is not complete before the age of 12 years. The relative absence of a myelin sheath coupled with the smaller nerve fiber diameter may explain why infants and children are subject to prolonged motor blocks with local anesthetic solutions of lower concentration [4]. In addition, the endoneurium of developing nerves is loose and easily penetrated by local anesthetic molecules in both directions. This may account for both the shorter onset and shorter duration of effect of local anesthetic action in younger age groups. Beyond infancy, the endoneurium contains more connective tissue, making it more difficult for the local anesthetic to traverse [9].
7.3 Pharmacokinetics
Pharmacologists and anesthesiologists share a common interest in pharmacokinetics, but rather than being concerned with volumes of distribution and total body clearance, the pediatric anesthesiologist wants to know how much local anesthetic can be safely administered to produce an effective nerve block in a timely fashion. However, these empirical and abstract concepts are not mutually exclusive.
Unlike other therapeutic agents administered to infants and children, local anesthetics can be delivered directly to their site of action. Traditionally, a large volume and high concentration of local anesthetic has been injected to ensure adequate anesthesia and analgesia. This was, in part, due to the relatively small number of local anesthetic molecules thought to reach the target nerves. The nerve sheath or perineurium is a very effective diffusion barrier. A large fraction of the delivered agent is absorbed by the surrounding tissue or is removed by the systemic circulation. Direct measurement in an animal model demonstrates that <2–3 % of an injected dose enters the target nerve. In addition, more than 90 % of an injected dose is taken up by the systemic circulation within 30 min of injection [11]. Ultrasound guidance may allow for more accurate deposition, necessitating a smaller volume and dose of local anesthetic.
Neonates and infants present significant pharmacokinetic peculiarities possibly leading to an increased risk of toxicity. Immature hepatic metabolism and marked differences in serum protein binding serve to increase serum concentrations of unbound amide local anesthetic.
7.3.1 Absorption
Regional anesthesia in the pediatric population involves the injection of a relatively large volume of a concentrated local anesthetic solution into a compact anatomical space. The rate of absorption into the bloodstream is a major determinant of systemic toxicity. This has been studied predominantly for epidural/caudal anesthesia. Explicit detail on the kinetics of other routes of administration is lacking for this population. What is evident, though, for both central and peripheral blocks, is the more rapid rate of absorption from the cephalic parts of the body [4]; for example, a cervical epidural leads to higher plasma levels of local anesthetic than a caudal epidural. Similarly, absorption decreases from head to foot for peripheral conduction and infiltration blocks due to the relative difference in vascularity between these areas.
7.3.2 Absorption from Epidural Space
The main component of the epidural space is fat, which is an important determinant of local anesthetic systemic uptake. More lipophilic local anesthetic molecules will be retained to a greater degree by epidural fat leading to subsequent delayed absorption. It has been demonstrated in adults that after a single-shot epidural injection, 30 % of a dose of lidocaine and 50 % of a dose of bupivacaine remained in the epidural space for 3 h after injection [12]. The time to maximum peak plasma concentration (Tmax) in infants for lidocaine and bupivacaine is 30 min after caudal or lumbar epidural injection [13]. Ropivacaine Tmax is longer in infants than in children and longer in children compared to adults. In children aged 1–2 years, ropivacaine Tmax is 115 min, whereas in children aged 5–8 years, ropivacaine Tmax is closer to the adult value of 30 [14]. The vasoconstrictive properties of ropivacaine may contribute to its prolonged absorption from the epidural space. After Tmax has been achieved, the rate of absorption slows down significantly so that it becomes longer than that of elimination, leading to a flip-flop effect in plasma drug concentration [4]. This continuous, protracted systemic absorption during the elimination phase, in combination with the buffering effect of plasma protein binding, limits the plasma concentration of unbound drug and is protective against toxicity.
7.3.3 Absorption from Other Routes of Administration
Absorption rates at different block sites are directly related to local blood flow and inversely related to local tissue binding [15]. As a consequence, plasma uptake is faster from the more vascular intercostal space or the axilla than from the caudal space. Vascular uptake of local anesthetic after intercostal nerve block occurs more rapidly than with any other regional technique [16]. Despite recently published epidemiologic data indicating up to a fivefold increase in the utilization of peripheral nerve blockade in the pediatric population, specific pharmacokinetic data are lacking [17]. Ilioinguinal-iliohypogastric nerve blockade is the only peripheral block whose kinetics have been reported with any degree of regularity. Significantly higher plasma concentrations of both bupivacaine and ropivacaine have been reported in one study following ilioinguinal-iliohypogastric nerve block as compared to caudal blockade [18]. Times to peak plasma concentration were much faster for both drugs following ilioinguinal-iliohypogastric nerve block. The narrow inter-fascial space at this block location may facilitate absorption. This, coupled with greater uptake of local anesthetic into epidural fat, may account for the lower plasma levels seen after caudal injection.
7.3.4 Distribution
Local anesthetics are distributed to the tissues and body fluid compartments after systemic absorption to the plasma. Volume of distribution (Vd) is a mathematical expression which depicts the distribution characteristics of a drug in the body. It is a measure of the degree to which a drug is delivered by the plasma to the organs and tissues of the body. Drugs with a small calculated Vd have a high concentration of drug in the plasma, have a concomitant low tissue concentration, and are more likely to accumulate to toxic levels. Drugs with a larger Vd are subject to slower elimination. Vd is influenced by degree of ionization/lipophilicity, plasma protein binding, and molecular size [19]. Since local anesthetics are weak bases that have dissociation constants (pKa values) above physiological pH, more than 75 % of the commonly used amide local anesthetic drugs exist in a hydrophilic ionized form at physiological pH (Table 7.1). Hence, they are highly water-soluble molecules but are less likely to cross lipid cell membranes. As a result of the delayed systemic absorption of local anesthetic drugs, it is impossible to calculate a volume of distribution with any accuracy. One study found bupivacaine to have a higher Vd in neonates and infants [20], which may be related to the higher fraction of unbound drug to plasma protein (see next section). Following adult epidural anesthesia, ropivacaine appears to have a smaller Vd than bupivacaine [21, 22], and indirect evidence points to a similarly reduced Vd in infants. Following single-shot caudal anesthesia, peak concentration of ropivacaine is higher than bupivacaine [23]. Two further studies have demonstrated that Vd of ropivacaine appears slightly smaller before the age of four [14, 24].
Local anesthetic is distributed to organs according to their vascular density. The local anesthetic is taken up within each organ according to the tissue-plasma partition coefficient (Table 7.3). The lungs play an important buffering role by taking the full impact of drug-laden venous blood. A variety of investigational techniques, including autoradiography, scintillation counts, and tissue assays, confirm the lung’s ability to quickly extract local anesthetic [25], although this buffering action of the lung is saturable.
Table 7.3
Tissue-blood partition coefficients (lidocaine)
Organ | Tissue-plasma λ | Tissue-blood λ |
---|---|---|
Spleen | 3.5 | – |
Lung | 3.1 | 5.4 |
Kidney | 2.8 | – |
Stomach | 2.4 | – |
Fat | 2.0 | 2.9 |
Brain | 1.2 | 1.7 |
Heart | 1.0 | – |
Muscle | 0.7 | 0.9 |
Liver | 0.6 | 2.9 |
Skin | 0.6 | – |
Bone | 0.4–0.9 | – |
7.3.5 Plasma Protein Binding
Local anesthetics bind tightly to serum proteins, greatly limiting the free fraction of available drug. This is clinically relevant as it is only the free or unbound fraction that is bioactive (i.e., readily available to cross cell membranes to become active at the sodium channel). Volume of distribution (see above) is inversely related to protein binding. Drugs which are highly protein-bound have limited passage into tissues resulting in a high drug plasma concentration and a low Vd. In adults, lidocaine is up to 70 % protein bound while bupivacaine, levobupivacaine, and ropivacaine are over 90 % protein bound [19].
Three principal blood components are involved in local anesthetic binding: the plasma proteins alpha-1-acid glycoprotein (AAG), human serum albumin (HSA), and erythrocytes. Like most weak bases, local anesthetics bind mainly to AAG. AAG has a greater affinity for binding local anesthetic by an order of magnitude of 5,000–10,000 compared to albumin [26]. Capacity for binding is relatively low, however, and saturation occurs at clinically relevant concentrations. Even though albumin is the most abundant plasma protein (50–80 times more abundant than AAG), it has a low affinity for amide local anesthetic drugs [4]. By virtue of its enormous binding capacity (it is almost unsaturable), together with its abundance, the role of HSA becomes significant when AAG is saturated.
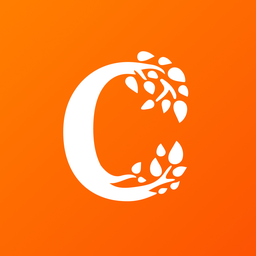
Full access? Get Clinical Tree
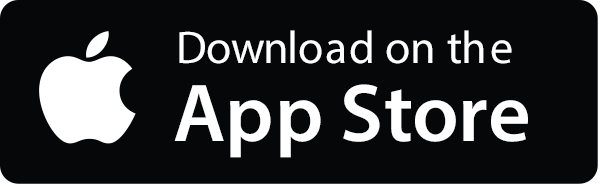
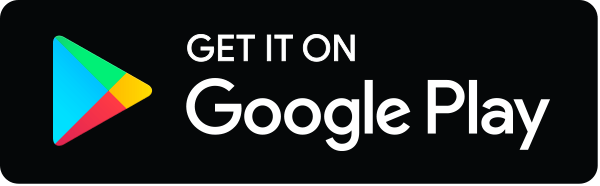