Parameter
Infant
Adult
Functional residual capacity
25–30 ml/kg
30 ml/kg
O2 consumption
6 ml/kg/min
3 ml/kg/min
CO2 production
6 ml/kg/min
3 ml/kg/min
Respiratory rate
35–50 breaths/min
12–20 breaths/min
Tidal volume
6 ml/kg
6 ml/kg
Alveolar ventilation
100–150 ml/kg/min
60 ml/kg/min
Vital capacity
35 ml/kg
60 ml/kg
Despite increased alveolar ventilation, neonates rapidly desaturate for two critical reasons. First, neonatal oxygen consumption is twice that of adults (6 ml/kg/min vs. 3 ml/kg/min), allowing for a high metabolic rate relative to FRC. This high metabolic rate in relation to the FRC leads to rapid desaturation in neonates and young infants. Secondly, infant closing volumes are high; hence small airways close during normal tidal breathing contributing to further desaturation.
Respiratory fatigue in neonates is a complex process that deserves further attention. Because the intercostal “accessory” muscles of respiration are not fully developed, respiratory work is primarily dependent upon the diaphragm. However, the neonatal diaphragm is comprised mostly of Type II fast-twitch fatigable fibers. In addition, neonates have noncompliant lungs but a very compliant chest wall, which make them prone to chest wall retractions, atelectasis, and increased work of breathing to maintain their functional residual capacity.
Adequate surfactant is critical for maintaining compliant lungs, open alveoli, and of FRC in compromised neonates at risk for respiratory fatigue/failure. Surfactant therapy can improve oxygenation and CO2 elimination in critically ill pediatric patients. Inadequate oxygenation and/or CO2 elimination, despite surfactant therapy, may require high-frequency oscillatory ventilation or even extracorporeal membrane oxygenation in select cases.
Cardiovascular
In utero, the high pulmonary vascular resistance allows for a parallel circulation, whereby both ventricles pump systemically with less than 10 % of the cardiac output entering the pulmonary system. To allow adequate placental oxygenated blood flow to the fetus, there are three shunts in utero: the ductus venosus, the foramen ovale, and the ductus arteriosus (Fig. 39.1). Oxygenated placental blood preferentially shunts across the foramen ovale because of relatively high right-sided heart pressures in utero (right to left shunt).


Fig. 39.1
Fetal circulation
At birth, the pulmonary vascular resistance drops with the expansion of both lungs, thus promoting pulmonary blood flow and elevating the PO2. As the left atrial pressure rises above right atrial pressure, the foramen ovale functionally closes; however, it still remains open in approximately 20 % of neonates. Upon exposure to higher PO2, the ductus venosus almost immediately closes, while the ductus arteriosus musculature constricts leading to closure in a majority of term neonates (may remain open in some premature neonates).
The neonatal ventricle is rather noncompliant with a steep pressure-volume relationship that resembles the elderly population (fixed stroke volume). Therefore, inadequate preload leads to a stiff underfilled ventricle and hence low systemic pressures. Although hypotension after anesthetic induction usually responds to volume administration, practitioners must avoid overloading these patients. Volume overload can lead to pulmonary edema because the left ventricular myofibrils have decreased contractile mass. Moreover, the sarcoplasm sequesters Ca2+ (ineffective Ca2+ adenosine triphosphatase activity), thus impairing myocardial contractility. In addition, the neonatal ventricle tolerates afterload poorly because of relative noncompliance and poor contractility.
The underdeveloped neonatal sympathetic nervous system leads to a predominance of parasympathetic tone, and hence neonates are more prone to bradycardia under hypoxic conditions. Cardiac output in neonates/infants is heart rate “dependent” because of the fixed stroke volume. With a resting heart rate of 120–160 bpm (Table 39.2), bradycardia (<80 bpm) significantly impacts the cardiac output.
Table 39.2
Cardiovascular parameters in children
Age | Blood volume (ml/kg) | Systolic BP (mmHg) | Heart rate (bpm) |
---|---|---|---|
Preterm neonate <37 weeks gestation | 90–100 | 50 | 120 |
Term neonate <1 month | 85 | 65 | 120 |
Infant (1–12 months) | 80 | 75–95 | 120–160 |
Children > 1 year | 75 | 75–95 | 80–100 |
Hematological
Circulating blood volume is highest for premature neonates (100 ml/kg), 85 ml/kg for term neonates, as compared with adults (70 ml/kg). Neonates have a hematocrit of about 60 %. The nadir in hematocrit occurs at about 6 months leading to a hemoglobin of 9 g/dl (hematocrit of 27 %). Because healthy neonates tolerate anemia better, blood transfusions may be deferred until the hemoglobin reaches 6–7 g/dl. However, the presence of congenital cyanotic heart disease or severe pulmonary disease has a higher trigger (Hb 10–13 g/dl) for blood transfusion. All neonates should receive irradiated blood to prevent graft-versus-host disease (where the donor lymphocytes attack the recipient’s bone marrow). Lastly, the platelet count should be greater than 50,000/mm3 to ensure the formation of an adequate primary hemostatic plug.
Renal
At birth, the GFR is 15–30 % of adult values (20–40 ml/min/1.73 m2 compared to 120 ml/min/1.73 m2) because of reduced nephron function, high renal vascular resistance, and low systemic perfusion pressures. In the 1st month, urine output is 1–2 ml/kg/h, but reduced GFR means large-volume administration can lead to hypervolemia and volume overload. Renally excreted drugs have a prolonged effect until the GFR approaches adult values at the end of 1 year. Because of decreased renal tubular function, neonates poorly concentrate urine as the medullary countercurrent exchange systems are immature. Both retention and excretion of sodium are impaired in neonates.
Neonates have proportionately a higher percentage of total body water (TBW) per kg body weight than adults, with fetuses having TBW of 90 %, preterm neonate 80 %, and full-term neonate 70 % of body weight. By 1 year, TBW decreases to the adult value of 60 % body weight. Initially, neonates have a predominance of extracellular fluid (ECF), which mobilizes as the GFR increases. Maintenance fluid requirements in neonates follow similar adult formulas; however, maintenance fluid should include sodium because of the reduced distal tubular reabsorption of sodium.
Temperature Regulation
Neonates and young infants have a large surface area to body weight ratio causing rapid heat loss in the operating room. The primary mechanisms for neonatal heat loss are conduction and radiation, with convection and evaporation playing a less significant role. Because neonates do not shiver, heat production occurs through non-shivering thermogenesis due to brown fat metabolism. Neonatal oxygen consumption is already elevated compared to adults (6 ml/kg/min vs. 3 ml/kg/min), and increased oxygen consumption due to cold stress may produce metabolic acidosis, cardiac irritability, or respiratory depression in compromised neonates. Heat loss can be prevented by using forced air-warming device, warm blankets, and warm intravenous fluids and most importantly by increasing the operating room temperature to 26 °C or higher. Heat lamp radiation is beneficial; however, it may produce mild burns when used too close to the skin.
Pediatric Airway
As compared to adults, the normal pediatric airway has a cephalad glottis (C4 vs. C6 in adults), large tongue, prominent occiput, long epiglottis, and short trachea and neck, with the narrowest part of the pediatric airway being the cricoid ring. Because neonates and infants <6 months are preferentially nasal breathers, bilateral choanal atresia can lead to complete airway obstruction. Airway management in healthy children is usually straightforward; however, airway management in craniofacial syndromes, such as Pierre Robin syndrome (micrognathia, retrognathia, and glossoptosis—large tongue in relation to oropharynx) or Treacher Collins syndrome (mandibular facial dysostosis with severe hypoplasia of the mandible and zygomatic arches), can be challenging.
Preterm Neonate
Neonates born of gestational age <37 weeks are labeled as preterm neonates. Preterm neonates have some unique differences than full-term neonates. Aberrant retinal vascular development leads to retinal tears and detachment and ultimately blindness, termed as retinopathy of prematurity (ROP). Risk factors for ROP include prematurity, low birth weight, and exposure to supplemental oxygen. Therefore, supplemental oxygen should be avoided, whenever possible, in neonates until 44 weeks of postgestational age and if given, should have a goal of maintaining oxygen saturation in the low 90 %.
Premature neonates are prone to the development of respiratory distress syndrome as the lungs mature after 34 weeks of gestation. Surfactant production begins at 26 weeks of gestation and enough is produced at 34 weeks of gestation for the alveoli to remain open. Neonates requiring supplemental oxygen at 36 weeks of gestation are said to have chronic lung disease. Premature neonates, because of reduced hepatobiliary function, are prone to hypoglycemia and hypocalcemia. Often they require dextrose-containing solutions for maintenance.
Preterm neonates are susceptible to apnea and thus require continous pulse oximetry monitoring in the post-operative period. Preterm neonates may have a hematocrit of 40 % as compared to 60 % for full term neonates, and this anemia further increases the risk of apnea. This predisposes a preterm infant to apnea. Moreover, preterm infants often have vitamin K deficiency and thrombocytopenia. Therefore, elective surgery should be deferred until 60 weeks postgestational age in preterm neonates. If surgery is performed before 60 weeks postgestational age, then these neonates are admitted postoperatively for observation for apneic episodes.
Pediatric Anesthetic Pharmacology
Inhalational Agents
The uptake and distribution of volatile anesthetics occur rapidly in pediatric patients. Firstly, high minute ventilation (high respiratory rate) to FRC ratio (5:1) causes a rapid increase in alveolar anesthetic concentration. Secondly, the distribution of high cardiac output to vessel-rich organs hastens the uptake of volatile anesthetics. The relatively insoluble sevoflurane (blood–gas solubility coefficient 0.62) has largely replaced halothane (blood–gas solubility coefficient 1.6) for inhalational induction. However, halothane’s safety is well established despite sensitizing the myocardium to catecholamines and directly reducing cardiac output.
Nitrous oxide use can diminish sevoflurane’s irritant properties and could hasten its uptake via the second gas effect; however, the clinical significance of this phenomenon is minimal. The cardiovascular effects of volatile agents include bradycardia and hypotension and could cause cardiac arrest in children less than 1 year of age and those with congenital heart defects. Cardiac arrests attributed to inhalational agents have declined since the decreased use of halothane.
The minimum alveolar concentration peaks in early infancy (2–3 months), remains elevated throughout childhood and adolescence, and then gradually declines with age. The MAC of sevoflurane peaks at 3.3 % for 3-month neonates, is 2.5 % for older children, and then declines to the 2 % value for adults. Intracardiac shunts (R > L shunt) may theoretically slow inhalational induction because greater blood flow bypasses the lungs. Conversely, L > R shunts would speed inhalation induction. The practical impact of shunt flow is negligible because the cardiac output drives anesthetic blood concentrations toward equilibrium.
Intravenous Induction Agents
Intravenous induction can be achieved with midazolam, ketamine, or propofol. Propofol is the commonly used intravenous agent for pediatric induction of anesthesia. Propofol doses of 2.5 mg/kg ablate eyelid reflexes within 50 s and increase mask acceptance. The maintenance of general anesthesia with intravenous propofol requires a continuous infusion of 200–300 mcg/kg/min. Prolonged infusion of propofol (in the ICU) may cause “propofol infusion syndrome” consisting of unexplained metabolic acidosis, heart failure, and even death.
Veno-irritation by propofol may be decreased by injection into a large antecubital vein and pretreatment or coadministration of lidocaine or opioids. In addition to causing profound apnea, propofol decreases both systemic vascular resistance and sympathetic outflow from the brain. While children experience mild reductions in blood pressure, preterm neonates with poorly developed sympathetics can become profoundly hypotensive with propofol.
The NMDA antagonist ketamine provides mild amnesia, profound analgesia, and produces general anesthesia at higher doses (1–2 mg/kg IV or 3–4 mg/kg IM). Although NMDA receptor blockade produces neuronal apoptosis and learning abnormalities in laboratory animals, ketamine’s long-term neurobehavioral effects on children are unknown. The benefits of ketamine include maintenance of spontaneous ventilation (does not typically produce apnea unlike propofol), bronchodilation while preserving airway reflexes, and activation of the sympathetic nervous system. The propensity toward secretions and post-op nausea/vomiting with ketamine is prevented with anticholinergics (glycopyrrolate/atropine) and antiemetics, respectively. Dysphoria is prevented with the coadministration of benzodiazepines (midazolam).
Opioids
The use of an opioid at induction allows decreased dosage of induction agent, attenuates sympathetic response to laryngoscopy, and prevents veno-irritation from propofol. Typical dosages of opioids in children are fentanyl 0.5–1 mcg/kg, sufentanil 1 mcg/kg, morphine 0.1 mg/kg, methadone 0.2 mg/kg, hydromorphone 10–20 mcg/kg, and remifentanil 1–2 mcg/kg. Morphine and methadone should be used with caution in infants <6 months of age because of impaired clearance. The rapid administration of high dosage of an opioid can cause chest wall rigidity making ventilation difficult. Chest wall rigidity is easily reversed by administering a non-depolarizing neuromuscular blocker or succinylcholine. The ultra-short-acting opioid remifentanil is ideal for procedural sedation, but its short duration of analgesia due to rapid nonspecific esterase metabolism prevents its use for postoperative analgesia.
Succinylcholine
Succinylcholine provides rapid onset of neuromuscular blockade within 30 s of administration (2 mg/kg IV or 4 mg/kg IM), allowing for rapid sequence induction when pulmonary aspiration of gastric contents is a concern. Common indications of succinylcholine use are small bowel obstruction, pyloromyotomy, trauma-induced delayed gastric emptying, emergency surgery with recent solid food ingestion, and the treatment of life-threatening laryngospasm (0.1 mg/ kg).
Succinylcholine is used cautiously in pediatric patients because it can cause bradycardia (even with a single dose), rhabdomyolysis, and hyperkalemic cardiac arrest in children with undiagnosed myopathy (Duchenne muscular dystrophy) and is associated with malignant hyperthermia. These lethal reactions prompted the FDA’s black box warning in the 1990s regarding the use of succinylcholine in at-risk pediatric populations. Other contraindications to succinylcholine include prolonged immobility, burn patients after 24 h, spinal cord injury after 24 h, myopathy, central core disease, and renal failure with hyperkalemia. Succinylcholine has been safely used in patients with cerebral palsy.
Non-depolarizing Neuromuscular Blocking Agents
Rocuronium (0.6–1 mg/kg—intubating dose) provides the onset of neuromuscular blockade within 1–2 min, and its paralytic effect is reversible in 30–45 min. Neuromuscular blocking agents have faster onset in children because of higher cardiac output and more rapid delivery to the neuromuscular junction. The ED-95 of a neuromuscular blocking agent is the dose providing 95 % single twitch depression. Multiple ED-95s (i.e., 2 mg/kg rocuronium) hasten the onset of laryngeal muscle relaxation by overwhelming the neuromuscular junction but at the expense of prolonged neuromuscular blockade. Rocuronium (with an ED-95 of 0.3 mg/kg) has a faster onset than vecuronium (ED-95 of 0.1 mg/kg). Both rocuronium and vecuronium are eliminated primarily in the hepatobiliary system (80 %), while the remainder of these drugs are excreted unchanged in the urine.
Pediatric Anesthesia Care
Preoperative Preparation
A parent signs the consent for anesthesia for children below 18 years of age. Children should be involved in the decision-making, as much as possible.
History and Physical
The preoperative history and physical should focus upon the birth history (prematurity or any complications), immunization status, any recent/current upper respiratory infection, and a pertinent review of systems (asthma, obstructive sleep apnea, cardiopulmonary symptoms). Unrepaired significant congenital heart disease, severe asthma, or moderate to severe obstructive sleep apnea likely precludes outpatient surgery and requires further evaluation.
Caution should be used for proceeding with elective surgery in healthy children recovering from a recent (2–4 weeks) upper respiratory infection (severe cough, high fever), understanding that these patients have a higher incidence of laryngospasm, bronchospasm, and oxygen desaturation. Risk factors for URI-related adverse events include airway surgery, history of prematurity, reactive airway disease, parental smoking, copious secretions, and nasal congestion.
Fasting
Pediatric fasting guidelines follow those for adults, namely, 6–8 h for solids, 6 h for formula, 4 h for breast milk, and 2 h for clear liquids. The incidence of pediatric aspiration is approximately 1:10,000 anesthetics, which is twice that reported in adults.
Preoperative Laboratory Tests
These are not routinely ordered for healthy patients undergoing minor surgical procedures. However, an electrolyte panel should be assessed in patients with renal disease and in patients with pyloric stenosis to determine the degree of resuscitation (chloride level > 95 meq/L). A preoperative CBC should be obtained for procedures involving significant blood loss, presence of coagulopathy, and presence of sickle cell anemia or if minor bleeding could be life-threatening.
Preoperative Sedation
The pediatric anesthesiologist plays a critical role in reducing both parental and patient preoperative anxiety on the day of surgery. Midazolam (0.25–0.5 mg/kg PO, maximum 20 mg) assists separation between the parent and the child, provides amnesia and anxiolysis, increases face mask acceptance, and smoothens the inhalational induction. Preoperative anxiety is often linked to postoperative delirium and maladaptive behaviors. Although the effectiveness of midazolam is well established, anesthetic induction relies upon trust and rapport between the child and the anesthesiologist.
A majority of pediatric patients over the age of 6–8 years tolerate mask induction well without the aid of midazolam. Also, midazolam is usually not administered to infants and neonates. Other adjuvants that can be administered preoperatively include IM ketamine (3 mg/kg) for combative patients, oral ketamine 4–6 mg/kg, and oral clonidine 4 mcg/kg. Rectal administration of premedication is less common, but historically rectal methohexital and thiopental have been used.
Anesthetic Induction
The induction of anesthesia in pediatric patients is done via the inhalational route or intravenous route. Children over 10 years are usually cooperative for intravenous insertion. The procedure for inhalation induction is described in the chapter of “Inhalational anesthetics.” If the patient is induced with an inhalational agent, an intravenous line is inserted once the patient is asleep (Table 39.3).
Table 39.3
Induction techniques in children
Inhalational induction | |
Face mask | May add flavor smell |
Induction | 10 l flow of 100 % oxygen or 70/30 N2O–O2, plus sevoflurane 6–8 % |
IV start | Once patient is asleep, no air bubbles in line |
N2O | Discontinued |
Prior to LMA insertion/intubation | Administer fentanyl or small dose of propofol if desired, and if intubating a non-depolarizing muscle relaxant (rocuronium), avoid succinylcholine (some do not use any muscle relaxant) |
Intravenous induction | |
Preoxygenation if possible, give propofol (1.5–2 mg/kg) or muscle relaxant if needed (rocuronium—0.6–1 mg/kg) | |
Endotracheal tube size and length | |
Premature | 2.5–3 mm tube |
Neonates | 3.0–3.5 mm tube |
Children tube size (diameter) formula | 4 + (age/4) in mm (correct size tube should have an air leak at 25 cm H2O pressure, additional size tubes 0.5 mm larger and smaller should be available |
Tube length formula | 12 + (age/2) in cm, correct length should be confirmed by auscultation |
Intravenous access in children may be challenging. For awake IV insertions, applying EMLA skin cream (eutectic 5 % mixture of local anesthetics—lidocaine and prilocaine 2.5 % each) 45 min prior to insertion may be beneficial. Locations for blind intravenous access in children include the saphenous vein (anterosuperior to the medial malleolus), median cubital vein (antecubital fossa), and the dorsal hand vein (between 4th and 5th carpal bones). For emergency purposes, intraosseous access may be obtained by inserting an intraosseous or Tuohy needle in the proximal tibia, distal tibia, or distal femur.
Special Pediatric Problems
Recent Upper Respiratory Tract Infection
Because children experience frequent upper respiratory tract infections, they often present for elective surgery with rhinorrhea, cough, and nasal congestion. When proceeding with elective surgery, consideration includes the complexity of surgery, family circumstances that may prevent rescheduling, and any coexisting disease that may increase the risk of anesthesia complications. After an upper respiratory tract infection, airway hyperresponsiveness persists for at least 2–4 weeks, but healthy children could be anesthetized albeit with an increased risk of bronchospasm, laryngospasm, and oxygen desaturation. Risk factors for anesthetic complications include history of prematurity, reactive airway disease, endotracheal intubation, parental smoking, and copious secretions.
Down Syndrome/Trisomy 21
Trisomy 21 affects 1.5/1,000 live births and is commonly associated with advanced maternal age. The characteristic features include flat facies, oblique palpebral fissures, simian crease, large tongue, short neck, and associated mental retardation. The presence of both a narrower trachea and smaller subglottis may require the use of a smaller size endotracheal tube than predicted. Obesity is common, which can make IV access difficult. Patients may have overreactive vagal tone, hypotonia, and joint laxity. Trisomy 21 children also have a higher incidence of duodenal atresia and cervical ligamentous laxity contributing to radiographic atlantoaxial instability, clinically the cervical spine IS stable. Granted there is controversy here but most folks agree that the c spine is stable.
The incidence of congenital heart disease is high (43 %), most commonly involving partial or complete atrioventricular canal and ventricular septal defects. These children have a propensity toward obstructive sleep apnea, pulmonary hypertension, and pulmonary infections. Enlarged tonsils and small ear canals necessitate frequent otolaryngology procedures, including tonsillectomy and myringotomy. Patients may also present for repair of cleft lip/palate, cataracts, and strabismus surgery.
Epiglottitis vs. Croup
Epiglottitis presents in children aged 2–6 years with fever, dysphagia, drooling, inspiratory stridor, and sitting forward to promote clearing of secretions. Epiglottitis is a life-threatening bacterial infection caused by Haemophilus influenzae type B (HIb). Vaccination has significantly decreased the incidence of HIb infection in the developed world. Radiographic studies should not delay airway management. A lateral neck X-ray, if taken, shows a thumbprint sign, representing a swollen epiglottis.
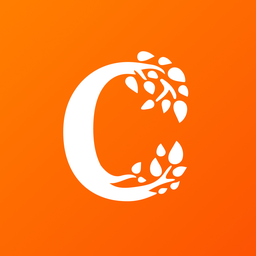
Full access? Get Clinical Tree
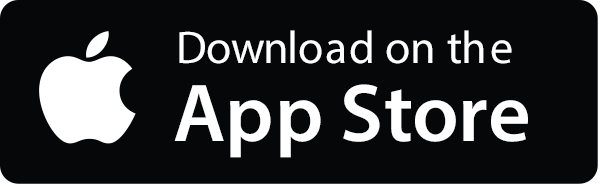
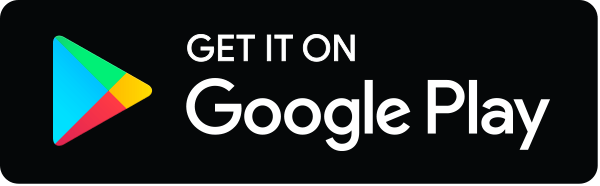