Key Points
- ▪
Congenital heart disease causes significant alterations in oxygenation, perfusion, and myocardial function after birth, and it can be categorized into hypoxic and normoxic lesions.
- ▪
The overall goal of therapy in shock is to treat the underlying cause, return adequate oxygen delivery to the tissues, and remove metabolic products that developed during anaerobic metabolism. It appears that the faster the body returns to adequate perfusion, the better the overall outcome.
- ▪
One of the specifics for neonatal resuscitation is the recommendation of positive pressure ventilation (PPV) with room air, unless chest compressions or medications are needed during the resuscitation, then the recommendation is still for PPV with 100% oxygen.
- ▪
Pediatric cardiac arrest is not a rare event. At least 16,000 American children (8-20 per 100,000 children per year) suffer a cardiopulmonary arrest each year.
- ▪
The four distinct phases of cardiac arrest and cardiopulmonary resuscitation (CPR) interventions are: (1) prearrest, (2) no-flow (untreated cardiac arrest), (3) low-flow (CPR), and (4) post-resuscitation and arrest.
- ▪
Acute respiratory distress syndrome (ARDS) diagnostic criteria have been revised recently and are now the Berlin Definition, where ARDS is separated into three mutually exclusive categories based on the degree of hypoxia. Mild is PaO <ce:inf>2</ce:inf> /FiO <ce:inf>2</ce:inf> = 201 to 300 with positive end-expiratory pressure (PEEP) > 5, moderate is PaO <ce:inf>2</ce:inf> /FiO <ce:inf>2</ce:inf> = 100 to 200 with PEEP > 5, and severe is PaO <ce:inf>2</ce:inf> /FiO <ce:inf>2</ce:inf> < 100 with PEEP > 10.
- ▪
Traumatic brain injury (TBI) is composed of two components—an initial primary injury owing to direct mechanical deformation of brain parenchyma and a subsequent secondary injury that can develop over hours to days. Secondary injury may be the result of multiple mechanisms including ischemia, excitotoxicity, metabolic failure and eventual apoptosis, cerebral swelling, axonal injury, and inflammation and regeneration.
- ▪
A vascular occlusive crisis in the lungs leads to acute chest syndrome (ACS). Acute chest syndrome is the leading cause of death and the second most common complication in sickle cell disease.
- ▪
Tumor lysis syndrome is a metabolic crisis precipitated by acute lysis of a large number of tumor cells. Serum uric acid, potassium, and phosphate concentrations are elevated. The elevated phosphate concentrations cause hypocalcemia.
- ▪
The role of family in the pediatric intensive care unit (PICU) has evolved over time, and the inclusion of family in the care of their child is now recognized as an important part of critical care.
- ▪
Accidents and trauma are the leading causes of death in children 1 to 14 years of age.
Acknowledgment
Portions of this chapter are taken from Dr. George Gregory’s fine treatment of this topic in the last edition.
Relationship Between the Intensive Care Unit and the Operating Room
The field of pediatric intensive care may have originated from anesthesia, but these areas have grown apart over time. Due to the extensive training, there are few individuals who cover both disciplines. With more complex patients, it is likely that care will occur both in the operating room and the intensive care unit (ICU). There needs to be excellent communication between the ICU and operating room clinicians to ensure a seamless transition of care. Many institutions require an attending to attending handoff between the ICU and anesthesia for each case. It is important that this occurs in the preanesthetic as well as the postanesthetic setting. Information regarding current ICU therapy response can simplify a potentially difficult anesthetic. Similarly, understanding the operative and anesthetic course will guide the next several days of management. A complete anesthesia sign-out includes pertinent past medical history, allergies, ease of mask ventilation, induction agents, ease of intubation, decisions regarding extubation, venous and arterial access, blood products, fluid totals, inotropic agents, medications delivered including timing of antibiotics, complications, laboratory values, and most recent blood gas. This information may be available in the anesthetic record; however, a short verbal summary by the anesthesiologist provides a greater amount of practical detail.
Family Partnered Care in the Pediatric Intensive Care Unit
The family is an important part of the critical care team and needs to be included in shared decision making. In pediatric hospitals, families participate in multidisciplinary rounds with their nurses, respiratory therapists, pharmacists, and physician caring for their child. This does not require more time than traditional rounds and it does not compromise teaching. There has been a significant push to increase family participation in both pediatric and adult ICUs. Family engagement is part of the ICU Liberation ABCDEF Bundle that is directed and supported by the Society of Critical Care Medicine. A great amount of information is available at www.iculiberation.org . An international multidisciplinary team of experts in neonatal, pediatric and adult critical care recently published Guidelines for Family Centered Care. The guidelines address the need for family presence in the ICU, the need for family support beyond the ICU; goals for communication, use of consult services such as Palliative Care and Ethics, and a means to address the operational and environmental issues in ICUs that prevent family engagement. We see significant family satisfaction with participation in rounds and we believe it likely benefits the team and patient as well. We anticipate a point in the future where we do not need to prove to anyone the need to have family involvement.
Giving the family a greater presence in the ICU with more responsibility and autonomy in decision making can increase their anxiety and distress. In addition to the potential development of posttraumatic stress disorder (PTSD) in our patients, parents of children admitted to an ICU can incur severe emotional distress. A recent review places the incidence of PTSD in parents of children in the PICU between 10% and 21%, with symptoms of PTSD occurring in up to 84% of families. PTSD can occur no matter how routine the caregivers may view the process. The ICU is a unique and often terrifying experience for families and children. The process of ICU care involves multiple caregivers, changing shifts, and endless physicians. For families in the ICU, there can be a loss of control, significant financial worries, and other factors that affect coping. Helping parents cope with their child’s critical illness and these stressors is a central part of intensive care. Parents may display behaviors that out of context may seem abnormal, such as excessive clinginess, intellectualizing the process, blaming others (including their spouses), minimizing, and seeking opinions everywhere (the internet, environmental care, etc.). We must attempt to understand what drives these behaviors to provide optimal care. We must help the parents be parents and educate them about their child’s illness. This emphasizes that social workers, psychologists, and child and family therapists are all part of the critical care team.
With the move to family-centered care, we must address the issue of parental presence during invasive procedures and cardiopulmonary resuscitation (CPR) efforts. There is increasing literature that families would like to have the choice to stay during CPR events or invasive procedures and the parents do find benefit to being present. We believe that allowing parents to stay during procedures or resuscitation is helpful for the parents coping with the trauma of a critically ill child. As each PICU addresses this issue there are several things to consider. Caregiver attitudes toward parental presence will need to be addressed, as the likelihood of this event increases over time. The decision to allow parental presence cannot be forced on providers. However, we have seen that resistance to family presence among providers is decreasing over time. A means for declining on the part of the clinician as well as the parent must be available. There must be someone identified who will stay with the family and support them. In our ICUs, this role been filled by social workers or members of the clergy. For those who are looking for assistance in making the transition to parental presence during CPR, there have been guidelines published from a national consensus conference. Parental presence during invasive procedures may pose a different challenge as these events occur more frequently compared with CPR. In the same manner, someone other than the person performing the procedure should be looking after the family, even for what we believe to be routine procedures. We also must give younger trainees the opportunity to opt out of family presence during procedures.
A final topic that needs to be addressed is the use of palliative care services for our critically ill patients. There is a role for early consultation of palliative care, as we do not believe in restricting its use or support to just those patients who are near to death. We feel that there is a significant benefit to early engagement for children who are at high risk for mortality during their hospitalization, for children with complex diseases, or those where they cognitive and physical abilities following ICU discharge will be significantly different than previously. There are great benefits to palliative care intervention to provide families ongoing support and opportunities to develop coping mechanisms. Many different PICUs have developed automatic triggers for palliative care consultation, so as not to miss opportunities to improve family support. Examples of triggers can be PICU duration, episodes of CPR, prolonged mechanical ventilation, and specific types of surgeries. A review by the IPAL-ICU (Improving Palliative Care in the ICU) Advisory Board in 2014 addresses the needs and goals of palliative care integration in the PICU.
Disclosure of Medical Errors
We believe it is ethically correct to disclosure medical errors to families. However, some clinicians may continue to resist due to concerns regarding litigation. In a survey of 1018 Illinois residents, 27% indicated they would sue, but 38% stated they would recommend the hospital if appropriate disclosure and remediation occurred. The conclusion drawn by the author of the study was that “[p]atients who are confident in their providers’ commitment to disclose medical errors are not more litigious and far more forgiving than patients who have no faith in their providers’ commitment to disclose.” Explanations of medical errors should come from the senior member of the team to the family. This is usually the current ICU attending, but can be the medical director of the ICU, based on the complexity of the incident and outcome. The discussion should include an explanation of what happened in layman’s terms, how it occurred, the repercussions and change of care planned for the child, and what will be done to prevent a similar error in the future. We find it helpful to have the ICU social worker present to help validate concerns and provide support. The attending remains present until all questions are answered or additional time is scheduled if necessary. Most hospitals will track errors or negative outcomes through a quality assurance program. Depending on the incident, a “root cause analysis” should be performed. Medical errors will occur, but they should also be an opportunity to improve practice quality and prevent future events.
In an ICU setting, there unfortunately will also be a need to cope with death and dying. Palliative care plays an important role when nothing medically can be done for the child. We also find their services exceedingly helpful for children with chronic medical conditions who are anticipated to die during a future admission. With a team approach, we try to minimize pain and suffering for the child and family at the end of life. Caregivers in and ICU must understand when to allow the families choices and support them over what may be their own beliefs and practices—as long as the goal remains to prevent further pain and suffering. The awareness of medical futility is increasing over time. However, with this concept there is a significant interplay with financial, societal, ethical, personal, and religious opinions and feelings. It may be difficult to define futility, but when the pain and suffering of continuing life are more severe than the inevitability of death, care may become futile. However, pain relief and caring support for the child and family can never be classified as futile care.
Organization of the Pediatric Intensive Care Unit
Medical and nursing directors, hospital administrators, and representatives from pediatric medicine, anesthesia, surgery, and the pediatric subspecialties should be responsible for policy and procedures pertaining to the PICU and should make recommendations regarding personnel staffing, equipment purchases, and structural and design changes within the unit. The medical director oversees the quality of patient care, patient triage, implementation of policy and procedures, in-service education, and coordination of consultants. Physician coverage should be full-time geographic at the resident, fellow, and attending staff level, and should include in-house, full-time coverage at night. The nursing director should have special skills in pediatric intensive care, education, and personnel management. The nursing staff must be trained in all aspects of pediatric critical care and resuscitation. Staffing should be flexible enough to provide one-on-one patient care when necessary. A multidisciplinary in-service program is essential for continuing education and orientation. Other team members include respiratory therapists, physical therapists, nutritionists, social workers, laboratory technologists, pharmacists, and psychiatrists and psychologists for the patients and staff. All medical and support personnel should be encouraged to participate in rounds, educational endeavors, and team meetings whenever possible. There must be adequate workspace around each bed and enough storage space to keep life support equipment within reach. Space for reading, meeting, sleeping, and showering should be available for the staff. Space should be provided for parents to remain with their children during the day and for parents to sleep overnight. Parents should be encouraged to participate as much as possible in the care of their child. Each bed space should be standardized so that it can be used to provide any level of care. Private rooms are ideal, but if shared rooms are necessary, the distance between beds must be adequate to ensure privacy and minimize nosocomial infection. Isolation rooms should be available within the confines of the unit. Devices for diversion and entertainment should be available for conscious children. Television and computer games are often better than heavy sedation. Adequate nurses and nursing involvement at the bedside will prevent potentially life-threatening events. Because sick children require close personal observation, centrally monitored nursing stations have little place in the PICU.
Cardiovascular System
Structural and Functional Development
The shape of the heart is complete by 6 weeks’ gestation, but myofibrillar density and maturation increase through the first year of postnatal life. During this time, myocytes engage in rapid protein synthesis and rapid cell growth, which requires a high intracellular concentration of nuclei, mitochondria, and endoplasmic reticulum. The greater number of nonelastic and noncontractile elements makes the neonatal myocardium less compliant, and it contracts less efficiently than the adult myocardium. In the fetus and newborn, the decreased ventricular compliance causes small changes in end-diastolic volume to induce large changes in end-diastolic pressure. In addition, augmentation of stroke volume by the Frank-Starling mechanism is less effective in young children. The newborn is more dependent on heart rate (HR) for maintenance of cardiac output. Cardiac output increases only about 15% with volume loading; it increases much more by increasing the HR. This is an important consideration when taking care of the critically ill infant.
Development of the Circulation
The adult and fetal circulation differs in many ways. The fetal circulation is distinguished by (1) the placenta as the organ of respiration, (2) high pulmonary vascular resistance (PVR), (3) low systemic vascular resistance (SVR), and (4) fetal ventricles that pump in parallel with right ventricular dominance. While the fetus lives in a low oxygen environment, the oxygen content of the blood of the fetus is similar to that of adults (20 mL of oxygen/100 mL of blood) because of a higher concentration of hemoglobin that has high affinity for oxygen. The neonatal circulation has several shunts—the ductus arteriosus, ductus venosus, and foramen ovale—that direct more oxygenated blood to the brain and heart and bypass the lungs. Changes then occur that allow the parallel circulation of the fetus to convert to the series circulation of the adult:
- 1.
With the first breath, expansion of the lung, increased alveolar oxygen, an increase in pH, and neurohumoral mediators and nitric oxide (NO) relax the pulmonary vasoconstriction.
- 2.
When the placenta separates from the uterine wall, the placental blood vessels constrict, and SVR and left ventricular afterload increase. The decrease in PVR plus increase in SVR raises left atrial pressure above right atrial pressure (RAP) and functionally closes the “flap valve” of the foramen ovale. The foramen ovale may not close anatomically for months to years, if ever. It is patent in at least 15% of adults.
- 3.
The decrease in PVR causes flow through the ductus arteriosus to reverse. This exposes the ductus to oxygenated systemic arterial blood, which along with the rapid decrease in prostaglandin E 2 after birth closes the ductus. Anatomic closure of the ductus requires several weeks.
- 4.
The ductus venosus closes passively with removal of the placental circulation and readjustment of portal pressure relative to inferior vena cava pressure.
- 5.
There is a further gradual decline in PVR secondary to structural remodeling of the muscular layer of the pulmonary blood vessels. During fetal life, the central pulmonary vascular bed has a relatively thick muscle layer. After birth, the muscle coat thins and extends to the periphery of the lung—a process that takes months to years to complete.
Development of Autonomic Control of the Circulation
The functional integrity of autonomic circulatory control during fetal and perinatal development is still a matter of considerable speculation. The fetal heart has reduced catecholamine stores and increased sensitivity to exogenously administered norepinephrine (NE).
Adrenergic innervation of the human myocardium is complete between 18 and 28 weeks’ gestation. Human newborns have low cardiac stores of NE and decreased numbers of sympathetic nerves after birth. Adrenergic responses are apparently present but diminished in newborn humans. In human neonates, the cholinergic system is completely developed at birth, and the heart is sensitive to vagal stimulation. Bradycardia is the probable response to an increase in autonomic tone. The baroreceptor reflex is present but incompletely developed at term in humans. In preterm infants, postural changes elicit no change in HR, suggesting an incomplete or attenuated baroreceptor response. The chemoreceptor response is well developed in utero. The fetal bradycardia that occurs in response to hypoxia is thought to be mediated through chemoreceptors and may be similar to the oxygen-conserving mechanisms of diving animals.
Myocardial Metabolism
Fetal myocardial metabolism differs from that of adults. Relative “hypoxia” is normal in utero, and infant hearts tolerate hypoxia better than the hearts of adults do. This difference may be due in part to high concentrations of glycogen in fetal myocardial tissue and to the ability to more effectively use anaerobic metabolism. Because of the high glycogen stores and the ability to use anaerobic metabolism efficiently, the fetal/newborn heart is relatively resistant to hypoxia and can be resuscitated more easily if oxygenation and perfusion are reestablished reasonably quickly.
Oxygen consumption increases precipitously after birth, presumably because neonates are required to maintain their own temperature. A full-term infant’s oxygen consumption in a neutral thermal environment is approximately 6 mL/kg/min; it increases to 7 and 8 mL/kg/min at 10 days and 4 weeks, respectively.
Common Cardiovascular Disease States
Congenital Heart Disease
Congenital heart disease causes significant alterations in oxygenation, perfusion, and myocardial function after birth ( Box 79.1 ). These abnormalities can be divided into hypoxic and normoxic lesions. The latter include obstructive lesions of the left side of the heart (mitral valve stenosis, aortic valve stenosis, aortic stenosis, anomalous pulmonary venous return, ventricular septal defect, or patient ductus arteriosus with a right-to-left shunt), whereas hypoxic lesions include tricuspid valve stenosis, pulmonary valve stenosis, pulmonary artery stenosis or aplasia, and the tetralogy of Fallot. Right-sided lesions cause hypoxia if the left-to-right shunting of blood is sufficient to cause congestive heart failure (CHF) and pulmonary edema. Newborns with significant congenital heart disease commonly have either cyanosis or CHF. The degree of dysfunction usually changes during the first few months of life as PVR decreases to adult levels. As PVR decreases, left-to-right shunting of blood usually increases, and the symptoms of CHF become more apparent. Many neonates with a significant ventricular septal defect, which may or may not be observed during the preoperative workup, have no left-to-right shunting for several weeks after birth; however, induction of alkalosis during surgery can increase shunting. In the newborn, the usual signs and symptoms of CHF include poor feeding, irritability, sweating, tachycardia, tachypnea, decreased peripheral pulses, poor cutaneous perfusion, and hepatomegaly. Many patients with pulmonary edema exhibit tachypnea without retractions. Cyanosis occurs with structural cardiac disease, but other causes such as respiratory disease, increased PVR (persistent pulmonary hypertension), and methemoglobinemia must also be considered. Congenital heart disease is diagnosed by physical examination, electrocardiogram (ECG), chest radiograph, and echocardiogram, postnatally and via fetal echocardiography. Cardiac catheterization is occasionally performed as interventional therapy or as a diagnostic tool. Magnetic resonance imaging (MRI) is used to define the anatomy of congenital heart lesions before cardiac surgery. Initial treatment of congenital heart disease is aimed at relieving CHF, improving systemic perfusion, and improving or maintaining pulmonary blood flow. The ductus arteriosus must be maintained open in instances of hypoplastic left heart syndrome, aortic stenosis or atresia, interrupted aortic arch, and symptomatic neonatal coarctation of the aorta. In many cases, infusion of PGE 1 sustains life until definitive surgical correction can be performed.
- 1.
Cyanotic congenital heart lesions
- ▪
Tetralogy of Fallot
- ▪
Transposition of the great arteries
- ▪
Hypoplastic left heart syndrome
- ▪
Pulmonary atresia with an intact ventricular septum
- ▪
Single ventricle
- ▪
Total anomalous pulmonary venous return
- ▪
Tricuspid atresia
- ▪
- 2.
Congenital heart lesions manifested as congestive heart failure
- ▪
Ventricular septal defect
- ▪
Patent ductus arteriosus
- ▪
Critical aortic stenosis
- ▪
Coarctation of the aorta
- ▪
Acute Circulatory Failure in Children (Shock and Sepsis)
Shock
Shock is the inability to provide adequate oxygen to the tissues that require it. The condition of shock depends on the balance of supply and demand of oxygen. Typically the body delivers excess oxygen to the tissues. Under periods of stress or illness there can be a decrease in supply caused by diminished blood flow or as decreased oxygen in blood. There can also be increased demand or oxygen extraction from the tissues. The content of oxygen is the blood is dependent on the amount bound to hemoglobin and the amount dissolved in plasma. Oxygen content (CaO 2 ) (mL/dL) = (1.34g/dL) (SaO 2 ) (Hb) + (PaO 2 ) (0.003). The normal oxygen content is approximately 20 mL/dL. Delivery of oxygen to the tissues depends on oxygen content and cardiac output. Oxygen delivery ( <SPAN role=presentation tabIndex=0 id=MathJax-Element-1-Frame class=MathJax_Error style="POSITION: relative" data-mathml='D˙O2′>[Math Processing Error]D˙O2
D ˙ O 2
) (mL/min) = oxygen content (CaO 2 ) × cardiac output (CO) × 0.01. Oxygen consumption ( <SPAN role=presentation tabIndex=0 id=MathJax-Element-2-Frame class=MathJax_Error style="POSITION: relative" data-mathml='V˙O2′>[Math Processing Error]V˙O2
V ˙ O 2
) is the demand portion of the equation. Oxygen consumption ( <SPAN role=presentation tabIndex=0 id=MathJax-Element-3-Frame class=MathJax_Error style="POSITION: relative" data-mathml='V˙O2′>[Math Processing Error]V˙O2
V ˙ O 2
) is independent of oxygen delivery ( <SPAN role=presentation tabIndex=0 id=MathJax-Element-4-Frame class=MathJax_Error style="POSITION: relative" data-mathml='D˙O2′>[Math Processing Error]D˙O2
D ˙ O 2
) above a critical threshold and over a wide range. Below this critical threshold <SPAN role=presentation tabIndex=0 id=MathJax-Element-5-Frame class=MathJax_Error style="POSITION: relative" data-mathml='V˙O2′>[Math Processing Error]V˙O2
V ˙ O 2
is dependent on <SPAN role=presentation tabIndex=0 id=MathJax-Element-6-Frame class=MathJax_Error style="POSITION: relative" data-mathml='D˙O2′>[Math Processing Error]D˙O2
D ˙ O 2
. For infants and young children, <SPAN role=presentation tabIndex=0 id=MathJax-Element-7-Frame class=MathJax_Error style="POSITION: relative" data-mathml='V˙O2′>[Math Processing Error]V˙O2
V ˙ O 2
is estimated at 175 mL/min/m 2 . Oxygen consumption is equal to oxygen delivery multiplied by oxygen extraction (O 2 EX) by the body: <SPAN role=presentation tabIndex=0 id=MathJax-Element-8-Frame class=MathJax_Error style="POSITION: relative" data-mathml='V˙O2=D˙O2×O2EX’>[Math Processing Error]V˙O2=D˙O2×O2EX
V ˙ O 2 = D ˙ O 2 × O 2 EX
. Oxygen extraction O 2 EX is equal to (CaO 2 – CvO 2 )/CaO 2 . CaO 2 is the oxygen content of arterial blood and CvO 2 the oxygen content of venous blood. The difference between oxygen content of arterial and venous blood is predictably 4 to 6 mL/100 mL blood. Initially, as oxygen delivery decreases, the oxygen consumption can remain constant via increased extraction. Below a critical threshold in oxygen delivery, oxygen consumption becomes dependent on delivery. When oxygen to meet metabolic needs of the body cannot be met, nonessential metabolism is decreased or eliminated. Such metabolism includes growth, neurotransmitter synthesis, thermoregulation, and so forth. In this way the remaining oxygen can continue to be substrate for mitochondria. There are organs in the body, such as the kidney, skin, intestines, and skeletal muscle, that receive a high supply of blood relative to their metabolic needs. These organs also have a high proportion of sympathetic nerve innervations that allow for redistribution of blood flow to organs that with limited oxygen reserves such as the brain and heart.
Classification of Shock
There are several schemas which clinicians use to classify shock. Further within these classification schemas, disease states can fall into more than one category. One classification schema separates shock into the categories of hypovolemic, cardiogenic, distributive or vasogenic, and extracardiac obstructive.
Hypovolemic shock can be due to hemorrhage from trauma or gastrointestinal (GI) losses from internal bleeding. Nonhemorrhagic hypovolemic shock can be due to external losses of fluid from vomiting, diarrhea, polyuria, and poor fluid intake. Fluid redistribution in cases of burns, trauma, and anaphylaxis can also be a cause.
Cardiogenic shock may be myopathic due to decreased heart function. For adults this may commonly follow myocardial infarction. For children, myocarditis or cardiomyopathy are more common. Other causes of cardiogenic shock include mechanical failure such as valvular regurgitation or obstruction. Significant arrhythmias may result in cardiogenic shock when contractions are so asynchronous they decrease cardiac output.
Extracardiac obstructive shock results from a physical obstruction that prevents adequate forward circulatory flow. Causes include inadequate preload secondary to mediastinal masses, increased intrathoracic pressure from tension pneumothorax, constrictive pericarditis, and cardiac tamponade from pericardial effusions. Pulmonary hypertension, pulmonary embolus, and aortic dissection can cause obstruction to systolic contraction.
Distributive shock is caused by a decrease in SVR and the maldistribution of end-organ blood flow. Cardiac output may be increased in distributive shock, however, blood pressure may remain low due to a very low SVR. Septic causes of distributive shock can be related to bacterial, fungal, viral or rickettsial infections or toxins produced from these infections. Toxic shock syndrome would be an example of toxin-mediated hypotension. Anaphylactic or anaphylactoid reactions are a type of distributive shock. Systemic inflammatory response syndrome (SIRS) may present with distributive shock. Spinal shock can result in distributive shock on a neurogenic basis. Adrenal insufficiency with low circulating hormones results in distributive shock decreased SVR.
Diagnosis of Shock
Maintaining a high index of suspicion is important to rapidly identify shock in pediatric patients. Volume losses may be readily apparent from the history of present illness. Fever, rash and irritability may point to infection. However, cardiogenic shock may present with vague reports of decreased activity and level of alertness. In addition, if the patient’s shock is currently compensated, changes in physical findings may be limited. A child in shock may present initially with tachycardia, cold extremities, and poor capillary refill. Further, in distributive shock, the child may be warm with just an isolated tachycardia. A brief pertinent physical exam should evaluate level of alertness, peripheral perfusion, mucous membranes, pulse rate and quality, respiratory effort, urine output, and blood pressure. In children, blood pressure may be preserved until the degree of shock has progressed. Hypotension is a sign of late and decompensated shock in children. Metabolic acidosis may not be present on the initial laboratory tests.
Compensatory Mechanisms
The body applies compensatory mechanisms with the onset of shock to maintain adequate tissue perfusion for as long as possible. There is redistribution of fluid from the intracellular and interstitium to the vascular space. There is a decrease in glomerular filtration to limit renal fluid losses. Renal fluid losses are also limited by the release of aldosterone and vasopressin. There is an increase in sympathetic activity and release of epinephrine. This results in decreased venous capacitance and some preservation of blood pressure. HR is increased as the body tries to maintain cardiac output. There is an increase in cardiac contractility through circulating catecholamines and adrenal stimulation. Increase in sympathetic nerve stimulation shunts blood away from nonvital organs. At the tissue level, transfer of oxygen from hemoglobin is increased by increased red blood cell (RBC) 2, 3-diphosphoglycerate, fever, and tissue acidosis.
Therapy and Outcomes
Aggressive therapy to treat pediatric septic shock appears to have resulted in improved outcomes. Therefore therapy for septic shock appears to be a good model for the treatment of shock in general. The overall goal of therapy in shock is to treat the underlying cause, return adequate oxygen delivery to the tissues, and remove metabolic products that developed during anaerobic metabolism. It appears the faster the body returns to adequate perfusion, the better the overall outcome. Many hospitals have developed sepsis pathways based on the data presented as follows that act as guidelines for resuscitation and are readily available to all care providers ( Fig. 79.1 ).

In 1991 Carcillo et al. described a population of 34 children that presented with septic shock to an emergency department. Shock was diagnosed based on hypotension for age, with decreased perfusion, poor peripheral pulses, cool extremities, and tachycardia. Sepsis was defined as a positive blood or tissue culture. Remarkably, within 6 hours of presentation, all the patients had a pulmonary artery catheter placed. The overall mortality for the group was 47%. However, in the nine patients who received more than 40 mL/kg of fluids in the first hour, there was only one death (mortality 11%). The authors point out this patient died with a second episode of sepsis 2 weeks later. In this study, the rapid fluid administration was not associated with an increase in cardiogenic pulmonary edema or ARDS.
In 2001 Rivers et al. published a study in adult patients with septic shock showing early, aggressive, goal directed therapy in the first 6 hours of care improved mortality. There were 263 adults were enrolled; 133 received standard therapy based on clinician discretion. The 130 patients randomized to early goal-directed therapy followed protocols treating hypovolemia and supporting blood pressure with vasoactive agents if necessary. The baseline characteristics of the two groups were similar. The in-hospital mortality was 46.5% in the standard therapy group and 30.5% in early goal-directed therapy group ( P < .01). Although in adults, this demonstrated the need for early aggressive intervention.
Following the Rivers publication, a task force was formed by members of the Society of Critical Care Medicine to address shock in children. Their work was published in 2002 as “Clinical Practice Parameters for Hemodynamic Support of Pediatric and Neonatal Patients in Septic Shock.” Their guidelines were incorporated into the American Heart Association’s (AHA) Pediatric Advanced Life Support (PALS) Provider Manual. Their guidelines were translated into Spanish and Portuguese and disseminated widely. The effectiveness of these interventions as well as an 2007 update was published by the same group in 2009. They highlighted significant improvements in mortality in dengue shock syndrome, malaria, and septic shock treated by community physicians using early goal directed therapy. The guidelines include rapid recognition of shock and early antibiotic administration and early administration of intravenous (IV) crystalloid. The initial resuscitation should include 20 mL/kg of isotonic saline or colloid pushed as a bolus to over 60 mL/kg until there is an improvement in the patient’s perfusion or rales or hepatomegaly develops. The goal is for the initial fluid resuscitation to occur in the first 15 minutes of therapy, and therapy should be initiated even if peripheral IV cannulation attempts fail, by placing and intraosseous (IO) device ( Fig. 79.2 ). The guidelines target therapeutic end points of normal pulses with no difference between peripheral and central; capillary refill ≤ 2 seconds; warm extremities, normalization of blood pressure for age, mental status, glucose concentration, ionized calcium concentration; and urine output greater than 1 mL/kg/h. If central venous access is not readily obtained, consideration should be given for placement of an IO line. Cold shock (cold mottled extremities with prolonged capillary refill) should be treated with dopamine up to 10 μg/kg/min and then epinephrine 0.05 to 0.3 μg/kg/min if there is no improvement. Warm shock (brisk capillary refill) should be treated with NE. Arrangements should be made early to admit the child to an ICU. If shock is not reversed with the inotropic support, hydrocortisone should be considered for catecholamine resistant shock. Recommendations for stabilization in the ICU following the first hour of therapy include monitoring central venous pressure, central venous saturation, and cardiac output. Persistent shock that is resistant to catecholamines should prompt the clinician to rule out pericardial tamponade, pneumothorax, or significantly elevated intra-abdominal pressure that may be compromising circulation. In the absence of a correctible condition, extracorporeal membrane oxygenation (ECMO) should be considered.

There were several new recommendations in the 2007 guidelines that addressed changes in the literature between 2002 and 2007. It was identified that the availability of skilled practitioners to place central venous access could delay the initiation of inotropic support. Therefore, the 2007 guidelines recommended the use of a peripheral IV dopamine or epinephrine if there was delay in obtaining central venous access. Ongoing monitoring of the access site should be performed. It was not recommended to use NE in a peripheral IV, due to risk of extravasation. In the 2002–2007 interval there were several pediatric and adult studies indicating adrenal suppression and increased severity of illness adjusted mortality with the use of etomidate. The 2007 guidelines do not recommend the use of etomidate unless it is in the format of a randomized controlled trial. Ketamine with atropine was recommended for sedation for invasive procedures in infants and children. However, due to limited experience, ketamine could not be recommended for the newborn population.
The 2007 guidelines recommend titrating therapy to cardiac output and indicate that there are several methods by which cardiac output can be measured. The use of pulmonary arterial catheters has decreased in pediatrics over time, but other methods are available. A good review of monitoring techniques was published by Mtaweh et al. in 2013. Cardiac output can be monitored by newer techniques analyzing the arterial pulse wave, transpulmonary thermodilution, carbon dioxide rebreathing, echocardiography, bio-impedance of the thorax, and ultrasound continuous-wave Doppler. These techniques are less invasive than pulmonary artery catheters. However, many still require validation studies in pediatric, and they may not be available at all centers.
One additional area to be addressed in the 2007 guidelines is in the area of fluid removal. A study published by Goldstein et al. in 2005 in pediatric patients with multiorgan failure, including acute renal failure requiring continuous renal replacement therapy (CRRT), showed improved survival in the group that had a lower percentage of fluid overload at the initiation of CRRT. While supporting the primary premise of fluid resuscitation, the 2007 guidelines offered new recommendation for fluid removal in patients with fluid overload and multiorgan failure. They recommended the use of diuretics, peritoneal dialysis, or CRRT in patients who had been adequately fluid resuscitated but were not able to maintain an even fluid balance through native urine output. Again, it should be noted that peritoneal dialysis and CRRT for pediatric patients may not be available at all centers. However, the association between fluid overload and mortality with acute renal failure has been seen in other studies and will likely be an ongoing issue in pediatric ICU care.
The concern for possible adrenal insufficiency during septic shock needs to be addressed by the clinician caring for the patient. There are certain instances where limited function of the adrenal axis is anticipated. This would include patients who have recently received glucocorticosteroids, ketoconazole, or etomidate. Further, patients with disease states such as purpura fulminans or those affecting the hypothalamus, pituitary, or adrenal glands will be at increased risk. Patients with adrenal insufficiency need supplemental corticosteroids. However, for children with septic shock but without these factors, it is not clear whether the risk of relative adrenal insufficiency or treatment with systemic steroids alter outcome. Dr. Zimmerman reviewed the adult and limited pediatric literature in 2007 for therapeutic steroid use in sepsis. He highlighted adult studies showing high dose short courses of steroids are associated with decreased survival. Further, data from the CORTICUS trial indicated that low dose steroids as a physiologic replacement during periods of vasopressor resistant shock resolve shock more quickly but there was no change in mortality. In turn, the 2007 guidelines were unchanged from 2002. Hydrocortisone treatment was only recommended for patients with absolute adrenal insufficiency or adrenal-pituitary axis failure and catecholamine-resistant shock. Absolute adrenal insufficiency was defined as peak cortisol concentration of less than 18 μg/dL obtained after corticotropin stimulation.
Cardiovascular Pharmacology
Pharmacologic support of the circulation includes positive inotropic and chronotropic agents, vasoconstrictors and vasodilators (afterload reduction), and antiarrhythmics (see Chapters 14, 18, and 86). Most currently used drugs have not been adequately tested in children, so dosage recommendations and anticipated effects must be extrapolated from adult doses and clinical experience.
Positive inotropic drugs are used to augment the cardiac output of patients with circulatory failure. Most inotropic agents also affect the HR and vasomotor tone. Tachycardia in a child is usually well tolerated and is frequently beneficial. In a neonate whose ventricles are relatively noncompliant and whose stroke volume is less variable, tachycardia is an important means of augmenting cardiac output. Because drugs that increase the HR or contractility also increase myocardial oxygen consumption, adequate arterial oxygenation and sufficient metabolic substrates are required when these drugs are administered. The cardiovascular response to sympathomimetic amines is attenuated in the presence of severe acidosis and possibly sepsis; higher infusion rates of these drugs are required and need readjustment as the acidosis improves. Commonly used inotropes are listed with brief comments regarding their use in pediatric intensive care are provided in the following paragraphs ( Table 79.1 ).
Drug | Effect | Dose (μg/kg/min) | Inotropy | Chronotropy | Vasodilation | Vasoconstriction |
---|---|---|---|---|---|---|
Epinephrine (Adrenalin) | α, β | 0.05-2.0 | ++ | ++ | ++ | |
Isoproterenol (Isuprel) | β 1 , β 2 | 0.05-2.0 | ++ | ++ | + | |
Dopamine (Intropin) | δ | 1-3 | +Renal splanchnic | |||
β > α | 5-15 | + | + | + or − | ||
β, α | >15 | + | + | + | ||
Milrinone | Bolus: 50 μg/kg over 15-min period | + | + | |||
Infusion: 0.375-0.75 | ||||||
Norepinephrine | α >> β | 0.05-1.0 | Slight+ | + | ++ | |
Nitroprusside | 0.5-10 | ++ | ||||
Arterial > venous | ||||||
Nitroglycerin | 1-20 | ++ |
Epinephrine
Epinephrine is useful for the treatment of shock in the presence of myocardial dysfunction. Typical starting doses in children are 0.05 to 0.2 μg/kg/min; with escalating doses up to 1 to 2 μg/kg/min, there is profound vasoconstriction in the periphery and abdominal organs to shunt blood to the heart and brain.
Dopamine
Dopamine is the most commonly infused inotrope in pediatric patients. Dopamine is the metabolic precursor of both NE and epinephrine. Its effects are dose dependent, with dopaminergic activity at low doses (although these low-dose dopamine effects have not been demonstrated in critically ill children); β-adrenergic activity with intermediate doses 5 to 10 μg/kg/min exhibiting chronotropic and inotropic effects; and some α-adrenergic activity at higher doses, with 10 to 20 μg/kg/min exhibiting peripheral vasoconstriction. Young children require higher doses of dopamine than adults do to produce the same effect. In one study, an infusion of 15 μg/kg/min was required to increase cardiac output above control levels after cardiac surgery. This may reflect the decreased releasable myocardial stores of NE in immature ventricles. Therefore, in the sick preterm infant there can be decreased dopamine clearance with a much greater vasopressor response than expected.
Vasopressin
Vasopressin is a pituitary peptide hormone with method of action on the kidney and vasculature. In the kidney, vasopressin controls water reabsorption in the renal tubules, and in the vasculature, it causes vasoconstriction by stimulating smooth muscle V1 receptors. Its clinical applications include GI hemorrhage, central diabetes insipidus (DI), and as a second- or third-line agent to treat hypotension.
Isoproterenol
Isoproterenol is a synthetic, potent, nonselective β-agonist with strong chronotropic effects with very low affinity to α-adrenergic receptors, and is usually well tolerated in children. However, high doses of isoproterenol can cause myocardial ischemia. Isoproterenol also induces vasodilation that is responsive to acute volume administration. It is often used for increasing HR in complete heart block, in the immediate postoperative period after cardiac transplantation to improve cardiac output by increasing HR in the denervated donor heart, and as a potent pulmonary vasodilator during pulmonary hypertensive crisis via β2-adrenergic receptor activity.
Dobutamine
Dobutamine provides positive inotropy and afterload reduction, β and α receptors. Its function is primarily as a inotropic agent but with less vasopressor activity compared with dopamine. It is only used as a continuous infusion of 5 to 20 μg/kg/min, and in some studies may increase myocardial oxygen In children but not in adults it causes tachycardia.
Norepinephrine
NE, a drug with strong α- and β-agonist effects, has had a resurgence of use in infants and children. Children with nearly normal cardiac function and marked peripheral vasodilation have good responses to this drug. It is especially useful in instances of warm septic shock, anaphylaxis, liver failure, and sympathetic blockade with regional anesthesia. It will increase SVR, but also limits mesenteric blood flow, including hepatic perfusion.
Milrinone
Milrinone is a selective phosphodiesterase III inhibitor that increases cyclic adenosine monophosphate by inhibiting breakdown. Milrinone has both inotropic and vasodilator effects, without acting on α and β receptors. This drug has improved the outcome of children who have low cardiac output syndrome after cardiac surgery. The loading dose of milrinone is 25 to 75 μg/kg administered over a period of 10 minutes; the maintenance infusion rate is 0.25 to 0.75 μg/kg/min. Loading doses are often avoided in the ICU setting because of resultant hypotension. Renal failure significantly increases the elimination half-life of this drug. Outside the cardiac ICU, milrinone is used for vasoconstricted septic shock and may have a role in the treatment of pulmonary hypertension.
Levosimendan
Levosimendan is a novel agent that increases the sensitivity of the contractile apparatus to calcium increasing inotropy by binding to cardiac myocyte troponin C. This agent will increase cardiac ejection fraction, while reducing catecholamine dose with minimal effects on blood pressure and HR. In children, the most common indications have been for cardiac failure or post–cardiac surgery, with a loading does of 612 μg/kg followed by an infusion of 0.1 to 0.2 μg/kg/min.
Nesiritide
Nesiritide is a recombinant form of the human B-type natriuretic peptide, the hormone release from the cardiac ventricles in response to volume overload and increasing mechanical wall stress. The action is on guanylate cyclase with resulting venous and arterial vasodilation. In addition, B-type natriuretic peptide leads to myocardial relaxation (lusitropy) and natriuresis. In children, it has been used to decrease central venous pressure and increase urinary output. Usual dosing suggestions for children and adults: initial 2 μg/kg bolus followed by a continuous infusion of 0.005 to 0.01 μg/kg/min.
Digitalis
Digitalis is useful for the long-term treatment of myocardial failure in children but may not be effective in neonates. Because of its long half-life and unpredictability, digitalis should be administered cautiously to children who have changing levels of serum potassium, calcium, and pH. In these cases, it is more appropriate to use rapid-acting, titratable inotropic agents.
Calcium
When serum ionized calcium levels are below normal, administration of calcium produces a positive inotropic effect. If the patient’s ionized calcium levels are normal, less marked inotropic effects occur. Low ionized calcium levels most commonly occur in patients with DiGeorge’s syndrome, when large volumes of citrate-containing blood products are rapid administered, and in neonates with relatively unstable calcium metabolism. Calcium also has effects on the cardiac conduction system. Rapid administration of calcium can cause severe bradycardia or asystole. This effect may be exaggerated in hypokalemic children or in those receiving digitalis. The vasomotor effects of calcium are controversial, but most reports show an increase in both SVR and PVR when the drug is administered.
Bicarbonate Therapy
Severe acidosis decreases myocardial function and tissue perfusion. Correction of acidosis with 1 to 2 mEq/kg of sodium bicarbonate is indicated for a pH below 7.20 if ventilation is adequate (PCO 2 <40 mm Hg if possible). Treatment is necessary because the circulatory system is refractory to sympathomimetic amines when the pH is less than 7.00. After initial correction of pH, persistence or reappearance of metabolic acidosis suggest a continuing underperfused state and the need for further therapy. Administration of sodium bicarbonate is only a stopgap measure to improve the response to drugs. Repeat infusions of sodium bicarbonate can cause hypernatremia and hyperosmolarity. Every 50 mEq of bicarbonate administered produces 1250 mL of CO 2 when the bicarbonate is fully reacted with acid. Consequently, adequate ventilation must be ensured while the drug is administered to avoid worsening the acidosis. Trishydroxymethylaminomethane (THAM) is an alternative to sodium bicarbonate, but larger volumes of THAM are required to produce the same amount of acid-base correction, which may be a problem in patients who have CHF. THAM does not increase PaCO 2 .
Vasodilators
Vasodilators are used to control systemic hypertension, increase cardiac output by decreasing afterload, control pulmonary hypertension, and control cardiac shunting. Vasodilators are an effective treatment of systemic hypertension and increase cardiac output in children with CHF. Treatment of pulmonary hypertension and intracardiac shunting with vasodilators has met with limited success because they decrease both PVR and SVR, which may increase extrapulmonary right-to-right shunting and further reduce pulmonary blood flow.
Nicardipine
Nicardipine is a dihydropyridine calcium channel-blocking agent used as an IV infusion that has powerful, antihypertensive effects in children. Studies have shown that the rapid onset of action is usually within 1 minute, adding to the profile appropriate for treating severe hypertension. Flynn et al. reports that nicardipine is an effective anti-hypertensive medication in children ranging in age from 2 to 18 years. In our institution, nicardipine is the drug of choice for hypertensive crisis. Infusion ranges: 0.5 to 1.0 μg/kg/min up to 3.0 μg/kg/min.
Sodium Nitroprusside
Sodium nitroprusside relaxes arteriolar and venous smooth muscle, which decreases afterload and preload. The half-life of sodium nitroprusside is only minutes, making it safe to titrate the drug to a desired effect. Nitroprusside is commonly used to control severe systemic hypertension, to provide controlled hypotension to reduce blood loss, and to increase cardiac output in children with low cardiac output syndromes (myocarditis, post–cardiac surgery status). Sodium nitroprusside can generally be used for days without problems, although cyanide and thiocyanate poisoning develops in some children, especially those with renal failure or reduced renal perfusion. Serum thiocyanate levels of 10 mg/dL are associated with weakness, hypoxia, nausea, muscle spasms, and disorientation. When these symptoms occur, nitroprusside administration should be discontinued immediately.
Hydralazine
Hydralazine is used to control systemic hypertension because it relaxes arterial smooth muscle more than it relaxes veins. Administration of the drug can cause headache, nausea, dizziness, sweating, and tremors. The most important acute side effect is tachycardia, which may increase cardiac output; labetalol, a β-antagonist, can counteract this effect.
Tolazoline and Phentolamine
These competitive α-adrenergic blockers have had varied success in the treatment of pulmonary hypertension. However, they are effective in treating the symptoms of pheochromocytoma preoperatively. Serious side effects of these drugs include tachycardia, ventricular arrhythmias, hypotension, and tissue edema.
Prostaglandin E 1
PGE 1 acts directly on vascular smooth muscle and has greatly improved the care of neonates with heart disease. At infusion rates of of 0.1 μg/kg/min, patency of the ductus arteriosus is maintained and a closed ductus reopens in some neonates. The drug is indispensable in the care of patients with ductus-dependent cardiac lesions, such as interrupted aortic arch, critical aortic stenosis, or hypoplastic left heart syndrome, where systemic blood flow is supplied through the ductus arteriosus. It is equally important for the care of patients who have pulmonary atresia or critical pulmonic stenosis. Apnea, fever, and hypotension are common side effects of this drug.
Nitric Oxide
NO is an endothelium-derived relaxing factor that selectively vasodilates the pulmonary vasculature. When administered by inhalation to patients with pulmonary hypertension, NO reduces PVR. It improves the survival of neonates who have reactive pulmonary hypertension. This compound is inactivated by hemoglobin before it reaches the systemic circulation. On rare occasions, NO causes systemic vasodilation or clinically significant methemoglobinemia when administered at 5 to 80 ppm.
Disorders of Cardiac Rhythm
Sinus tachycardia or an elevated HR for age is not considered an arrhythmia. However, patients with a significantly increased HR may be the most critically ill in the ICU. Causes of tachycardia include hypovolemia, fever, pain, anxiety, CHF, myocardial disease and dysfunction and thyrotoxicosis. With all of these causes, the goal is to treat the underlying disease state and not the tachycardia. For children without underlying heart disease, temporary increases in HR up to 180 to 200 beats/min is well tolerated. This is also not uncommon; children cannot increase their stroke volume so they increase their cardiac output by increasing their HR. Again, the goal is not to specifically control an elevated HR but to treat the cause of the tachycardia. Sinus arrhythmia is a phasic acceleration and slowing of the HR that occurs with respiration. This is not an uncommon finding. It indicates that the patient has a vagal tone greater than sympathetic tone and it can indicate that there is good cardiac reserve. A slow HR or sinus bradycardia is another relatively common heart rhythm seen in the ICU. It is an unremarkable finding in an older teenage patient who is relatively fit. Other potential causes can include increased intracranial pressure (ICP), hyperkalemia, hypothermia, profound hypoxia, and hypothyroidism. These causes should also be investigated. A slow HR is being seen more commonly with the increased of Dexmedetomidine but can also occur with beta blockers or Digoxin use. Sinus node dysfunction can occur following repair of congenital heart disease in children. Temporary slowing may be treated with the transcutaneous pacemaker placed during surgery. In the absence of myocardial pacing, if there is complete heart block or a very slow ventricular escape rate, a pacemaker may be needed shortly after the cardiac surgery. Otherwise, some amount of time is given to see if this will resolve.
Normal cardiac conduction starts in the sinus node. The electrical activity propagates through the internodal pathways in the atrium, is delayed in the AV node, it travels though the bundle of His, and then is conducted to the ventricles through the left and right bundle branches. Supraventricular tachycardia (SVT) is an elevated HR occurring at the level of the atrium, the AV node, or both. SVT typically has a narrow QRS morphology. Sinus tachycardia is therefore not a type of SVT but an acceleration of the normal conduction pathways. SVT includes reentrant and non-reentrant tachycardias.
The reentrant tachycardias include AV node reentrant tachycardia (AVNRT), orthodromic reciprocating tachycardia (ORT), and atrial flutter. AVNRT is what is classically thought of as pediatric SVT. The reentrant tachycardias occur due to the presence of an accessory conduction pathway that allows for abnormal electrical conduction in the heart. The presence of the abnormal pathway may be apparent on a standard ECG such as the case of Wolf-Parkinson-White (WPW). Alternatively the abnormal pathway may not appear on an ECG and is described as a concealed pathway. Concealed pathways are non-WPW ORT. In AVNRT, the AV node itself is the area in which the reentrance occurs. In atrial flutter there is a micro-reentrant circuit within the atrial tissue itself. In children, the circuit is typically near the tricuspid valve. In atrial flutter, after the reentrant circuit in the atria, the conduction proceeds through the AV node, where it is slowed. The reentrant circuit is small, and the rates of atrial flutter can be very high. As the conduction is slowed in the AV node, these high rates are not usually conducted to the ventricle. However, if atrial flutter or fibrillation occurs in a patient with WPW, the accessory pathway can allow conduction of the electrical impulse at a rate much greater than through the AV node. This can lead to ventricular tachycardia or fibrillation and can cause sudden death.
The non-reentrant causes of SVT occur due to abnormal automaticity of myocardial tissue. Causes of abnormal automaticity include atrial fibrillation and ectopic atrial tachycardia (EAT). In non-reentrant SVT, the elevated atrial rate is slowed as conduction goes through the AV node. In children, atrial fibrillation is caused by disorganized circuits typically near the pulmonary veins. This rhythm is described as irregularly irregular. EAT is rapid atrial beats that are consecutive and occur without sinus morphology. There can be one focus of the rapid atrial beats. Alternatively, in multifocal or chaotic atrial tachycardia, there can be several different atrial origins. Brief periods of EAT usually do not cause much sequel but can lead to cardiomyopathy if it is prolonged.
Treatment of reentrant SVT is based on whether the patient is clinically unstable or stable. The abnormal reentrant circuit can be interrupted with synchronized cardioversion or other methods. If the patient is unstable, reentrant SVT is treated with synchronized cardioversion with a dose of 0.5 to 1 J/kg. If the patient is stable, there is time to try other therapies. Therapies that increase vagal tone such as ice to the eyes or a Valsalva maneuver may interrupt the reentrant circuit. The medication adenosine will temporarily block conduction through the AV node. Adenosine can be used to interrupt episodes of reentrant SVT that have a reentrant circuit using the AV node. If the reentrant circuit does not include the AV node adenosine might not stop the tachycardia, but it may be helpful with diagnosis. Following administration of adenosine, there will be a period of sinus pause. Adenosine is metabolized by erythrocytes so it is a short acting medication. Equipment to perform cardioversion should be immediately available when adenosine is given. The initial dose is 0.1 mg/kg, and the dose should be given quickly with a sufficient flush. It is more effective when given centrally if available. If it is not effective at the dose of 0.1 mg/kg, the second dose can be increased to 0.2 mg/kg. Higher doses than this are not likely to be more effective, and if the SVT persists, other medications such as amiodarone, procainamide, or verapamil may be necessary. Amiodarone can block an accessory pathway as well as the AV node. If given too quickly, amiodarone will decrease the blood pressure. For both amiodarone and procainamide, continuous infusions may be necessary after the loading dose. Verapamil will block the AV node for a much longer period of time than adenosine. However, in younger patients (<2 years), verapamil may induce other life-threatening arrhythmias. If a patient has SVT, a cardiology consult should be obtained. This is because an echocardiogram may be beneficial, and depending on the cause, there may be a need for long-term follow-up.
Junctional ectopic tachycardia is caused by abnormal automaticity in an area around the atrioventricular junction. This is not a common pediatric arrhythmia but can occur following repair of congenital heart disease. The most common lesion with which this occurs is tetralogy of Fallot.
Wide complex tachycardias are assumed to arise from the ventricle until proven otherwise. SVT can cause a wide complex tachycardia if there is aberrancy of the conduction through the pathways in the ventricle. However, given the risk of delaying therapy, all wide complex tachycardias should initially be treated as ventricular tachycardia. If there is no pulse, initiate CPR, defibrillate, and follow PALS guidelines. If the patient has a pulse and stable blood pressure, there may be time to consider other therapies. These therapies are cardioversion or use of medications such as adenosine, amiodarone, or procainamide. Ventricular fibrillation is treated with CPR, defibrillation, and then medications following PALS guidelines. Ventricular rhythms should be quickly examined for the possibility of Torsades de Pointes, as giving magnesium will be especially helpful.
In the course of following the continuous rhythm strip of all children in the PICU, common abnormalities may be noted. A prolongation of the PR interval or first degree heart block can occur in otherwise normal children. Typically, these children are asymptomatic. Second-degree heart block occurs as Mobitz Type I and Mobitz Type II. Mobitz Type I is also known as Wenckebach . This is a gradual prolongation of the PR interval until a QRS is not seen and then the cycle restarts. This occurs due to delay of the electrical signal in the AV node, and it can be a benign phenomenon. Mobitz Type II is less likely to be a benign phenomenon. The PR interval remains constant, but intermittently there is no QRS or ventricular beat. This phenomenon is evidence of disease of the His-Purkinje fibers and can progress to complete heart block. Mobitz Type II occurs much less frequently in children as compared with adults. Complete heart block or third-degree AV block is the complete dissociation of atrial and ventricular activity. In complete heart block, the atria contract at a rate greater than the ventricle. Ventricular contraction occurs through ventricular escape. Congenital complete heart block can occur in infants born to mothers who have an autoimmune disorder such as lupus. When there is damage to the conduction pathway during surgery for congenital heart disease, complete heart block can occur. As immediate treatment of complete heart block, the ventricular rate may be increased with IV isoproterenol. When this is ineffective, transthoracic or transvenous pacing will be necessary until definitive therapy can be arranged.
Premature beats are also seen quite frequently in a PICU setting. Premature atrial contractions are usually benign and are caused by automaticity of atrial tissue other than the sinus node. Premature ventricular contractions (PVC) are usually benign with a few considerations. The presence of a central venous catheter touching the heart may cause increased PVCs, and if present, the catheter should be pulled back. Electrolyte abnormalities; typically of potassium, magnesium, and calcium, may cause PVCs. The frequency of PVCs may improve as the electrolytes are corrected. Exogenous catecholamines may cause PVCs, and the frequency of PVCs may improve if the catecholamines can be decreased. Endogenous catecholamines may cause PVCs, and the frequency may be decreased if pain or anxiety is treated.
Hypertension
Essential hypertension is uncommon in children, but when it occurs, it is often associated with another disease process ( Box 79.2 ) and is frequently difficult to control. The acute onset of severe systemic arterial hypertension is a medical emergency that has the potential of causing cardiovascular decompensation, encephalopathy, seizures, and intracranial hemorrhage. In older children, the neurologic manifestations of hypertension are more likely to precede cardiovascular decompensation. Neonates with severe hypertension are frequently initially found to have CHF. Treatment of hypertension is tailored to the disease process, the absolute degree of hypertension, and the presence of cardiovascular or neurologic symptoms.
Renal
Acute glomerulonephritis (e.g., poststreptococcal, Henoch-Schönlein purpura)
Hemolytic-uremic syndrome
Chronic glomerulonephritis (all types)
Acute and chronic pyelonephritis
Congenital malformations (dysplasia, hypoplasia, cystic diseases)
Tumors (e.g., Wilms, leukemic infiltrate)
Post–renal transplantation status; also rejection
Oliguric renal failure
Trauma
Obstructive uropathy
After genitourinary surgery
Blood transfusions in children with azotemia
Cardiovascular
Coarctation of the aorta
Renal artery abnormalities (e.g., stenosis, thrombosis)
Takayasu’s disease
Endocrine
Pheochromocytoma
Neuroblastoma
Adrenogenital disease
Cushing syndrome
Hyperaldosteronism
Hyperthyroidism
Hyperparathyroidism
Iatrogenic
Intravascular volume overload
Sympathomimetic administration (e.g., epinephrine, ephedrine)
Corticosteroid administration
Rapid intravenous infusion of methyldopa
Miscellaneous
Immobilization (e.g., fractures, burns, Guillain-Barré syndrome)
Hypercalcemia (e.g., hypervitaminosis D, metastatic disease, sarcoidosis, some immobilized patients)
Hypernatremia
Stevens-Johnson syndrome
Increased intracranial pressure (any cause)
Dysautonomia
After resuscitation
Neonatal Resuscitation
Profound changes occur in the cardiovascular and respiratory systems at birth. Failure to successfully make these changes may result in death or central nervous system (CNS) injury. Consequently, someone capable of performing neonatal resuscitation must be present at every delivery. Wasting time finding someone to resuscitate the neonate may be disastrous for the infant. This section discusses the causes and effects of cardiorespiratory insufficiency at birth and the techniques of resuscitation. When possible, the recommendations of the American Academy of Pediatrics have been followed.
Guidelines for neonatal resuscitation have been issued by many organizations, including the AHA and the American Academy of Pediatrics.
Initial Assessment of the Fetus at Birth
Initial stabilization should begin with a rapid evaluation of the newborn to determine if the infant is term, breathing, or crying, and has a normal tone ( Table 79.2 ).
Clinical Condition | Intervention |
---|---|
Initial resuscitation | Clear infant airway Warm, dry, stimulate, position Evaluate HR, respirations, color |
HR > 100 beats/min, breathing, no cyanosis | Observation |
HR > 100 beats/min, but persistent respiratory distress or cyanosis | Clear airway SpO 2 monitoring Consider CPAP |
Apnea, gasping, or HR < 100 beats/min | Bag-mask PPV SpO 2 monitoring |
After initiation of resuscitation (PPV), HR > 100 beats/min, effective ventilation | Post-resuscitation care |
HR < 60 beats/min | Consider intubation Chest compressions Coordinate PPV |
HR = 60-100 beats/min | Continue with PPV SpO 2 monitoring |
Ongoing Assessment
Ongoing assessment consists of three signs: HR, respirations, and oxygenation. The preferred method for auscultation of HR is by auscultation. All of these vital signs should be determined within the first 30 seconds.
Clearing The Airway
Proper positioning by placing the infant in the sniffing position is recommended, and the practitioner must try to avoid either underextension or hyperextension, both of which will obstruct the airway. Deep sucking should be avoided even in healthy, vigorous newborns, because of risks of vagal-mediated bradycardia. This does not apply to newborns who may have airway obstruction or the depressed infant with meconium (covered later in this section.)
Temperature Control
During the initial resuscitation period, the goal temperature for the newborn is normothermia. The initial step is to dry the infant and warm the infant to a goal axillary temperature of 36.5°C. The goal for each neonate is euthermia. Infants wrapped in polyethylene from the neck down will avoid evaporative heat loss. Controlled hypothermia should only be attempted in select tertiary centers within hours after birth in infants with hypoxic-ischemic encephalopathy (HIE).
Oxygen
One of the recent changes in neonatal resuscitation in the 2011 Neonatal Resuscitation Program Guidelines is the recommendation of positive pressure ventilation (PPV) with room air, unless chest compressions or medications are needed during the resuscitation then the recommendation are still for PPV with 110% oxygen. It is important to place a preductal (right hand) oximeter probe on the newborn if PPV is initiated. For the preterm infant, oxygen should be blended to goal saturation targets. In summary: (1) Use room air in the baby is cyanotic or needs PPV. (2) If the baby is less than 32 weeks, titrate oxygen ( Table 79.3 ). (3) Use 100% oxygen if chest compressions or medications are given, then titrate to targeted SpO 2 . (4) Apply oximeter to right hand (preductal).
Time after Birth (min) | Target SpO 2 (%) |
---|---|
1 | 60-65 |
2 | 65-70 |
3 | 70-75 |
4 | 75-80 |
5 | 80-85 |
10 | 85-95 |
Ventilation
Breathing usually begins by 30 seconds after birth and is sustained by 90 seconds of age. A few minutes after birth, the respiratory rate (RR) of normal neonates is between 40 and 60 breaths/min. The absence of a pause between inspiration and expiration helps develop and maintain functional residual capacity (FRC). Apnea and bradypnea prolong exhalation, reduce FRC, and cause hypoxia. Causes of apnea and bradypnea include severe acidosis, asphyxia, maternal drugs, infections, and CNS damage. Tachypnea (>60 breaths/min) occurs with hypoxemia, hypovolemia, metabolic and respiratory acidosis, CNS hemorrhage, pulmonary gas leaks, pulmonary disease (e.g., hyaline membrane disease, aspiration syndromes, infections), pulmonary edema, and maternal drugs (e.g., narcotics, alcohol, magnesium, barbiturates).
Recommendations now are that initial breaths should be at 20 cm H 2 O. Ventilation should be performed at 40 to 60 breaths/min with reassessment of HR, color, and breath sounds. In the neonate, rising HR may be the best assessment of adequate ventilation. If gastric distention becomes a problem, hindering compliance, a gastric tube may be placed (8 Fr) to improve compliance. Both sides of the chest should rise equally and simultaneously with inspiration, but the amount of rise should not exceed that associated with the neonate’s normal spontaneous breathing. The presence of breath sounds may be misleading because they are well transmitted within the neonate’s small chest. A difference in breath sounds between the two sides of the chest should raise suspicion of endobronchial intubation, pneumothorax, atelectasis, or a congenital anomaly of the lung. The presence of loud breath sounds over the stomach suggests esophageal intubation or a tracheoesophageal fistula. If ventilation is adequate, the neonate will become pink, initiate rhythmic breathing, and have a normal HR.
Because most asphyxiated neonates have no lung disease, they can be effectively ventilated with peak airway pressures lower than 25 cm H 2 O, even for the first few breaths. Those with stiff lungs (e.g., erythroblastosis fetalis, congenital anomalies of the lung, pulmonary edema, severe meconium aspiration, diaphragmatic hernia) may require higher inspiratory pressure to ventilate their lungs and are more likely to have pulmonary gas leaks. To reduce this likelihood, the lungs should first be ventilated with an inspiratory pressure of 15 to 20 cm H 2 O and inspiratory rate of 150 to 200 breaths/min. If low-pressure (low-volume), high-rate ventilation does not improve the oxygenation, higher pressure and volume may be required. Failure to adequately ventilate the lungs at birth may worsen hypoxemia and lead to CNS damage or even death. If P a O 2 exceeds 70 to 80 mm Hg or SaO 2 exceeds 94%, the inspired oxygen concentration should be reduced (if increased concentrations of oxygen are used) until SaO 2 and PaO 2 are normal for age. Oxygenation is maintained at the low range of normal in neonates 34 weeks’ or less gestation to avoid the retinopathy of prematurity. The neonate’s HR should be monitored continuously during endotracheal intubation because the process of tracheal intubation may cause arrhythmias in hypoxic neonates.
If the practitioner is having difficulty with bag mask ventilation or fails intubation, a laryngeal mask airway (LMA) should be considered.
Pneumothorax
Pneumothorax occurs in 1% of all vaginal deliveries, in 10% of meconium-stained neonates, and in 2% to 3% of neonates who require mechanical ventilation in the delivery room. The hemithorax containing free air is usually hyperexpanded and moves poorly with ventilation. The point of maximum cardiac impulse is shifted toward the side without the pneumothorax. Heart tones may be muffled.
If a small, high-intensity cold light is placed directly on the skin of the neonate’s chest, the involved side of the chest will glow if a pneumothorax is present. Pneumothoraces are relieved by needle or chest tube drainage.
Endotracheal Intubation
The head should be placed in a neutral or “sniffing” position during bag-and-mask ventilation and tracheal intubation. An appropriately sized endotracheal tube (ETT) is inserted and its tip is placed 1 to 2 cm below the vocal cords, depending on the size of the neonate. Usually, this means that the distance from the tip of the tube to the gums is 7, 8, 9, or 10 cm in 1-, 2-, 3-, and 4-kg infants. A small gas leak should be present between the ETT and trachea when the ventilation pressure is 15 to 25 cm H 2 O. This usually entails the use of a 2.5-mm (internal diameter) tube for neonates weighing less than 1.5 kg, a 3.0-mm tube for those between 1.5 and 2.5 kg, and a 3.5-mm tube for those weighing more than 2.5 kg. Successful tracheal intubation is confirmed by observing the ETT pass through the vocal cords, by observing bilateral chest movement with each mechanical inspiration, and by observing condensation in the ETT during exhalation. Breath sounds should be much louder over the chest than over the abdomen, and the skin color, HR, and SaO 2 should improve with positive-pressure ventilation. Carbon dioxide should be present during exhalation. However, the small tidal volumes and low pulmonary blood flow of some infants at birth may make it difficult to use capnography effectively.
Cardiac Compressions
Place both thumbs on the sternum and allow the fingers to support the back ( Fig. 79.3 ). Compress the sternum to approximately one-third the depth of the chest. Three compressions should be performed with a breath in place of the fourth compression for an effective compression rate of 90 compressions and 30 breaths/min. HR should be evaluated every 45 to 60 seconds, and if after adequate ventilation and compressions for 60 seconds the HR is still less than 60 beats/min, then medications should be considered.

Medications
Resuscitation with medications are needed only for the infant that is critically depressed or presents with significant anomalies leading to cardiovascular depression. There should be a quick reference drug list designed for each delivery room for easy access for these rare occasions, and should help with dosing based on an estimated weight of the infant at birth. IV route of administration is the preferred for administration of resuscitation medications; however, IO and umbilical venous catheters can be placed rapidly by trained individuals and may be life-saving.
Epinephrine
The primary medication used in the resuscitation of a newborn is epinephrine, and should be given if the infant’s HR is less than 60 bpm, 45 to 60 seconds after the initiation of PPV and chest compressions. The recommended dose is 0.1 to 0.3 mL/kg of 1:10,000 concentration; (0.01-0.03 mg/kg), followed by a 1 mL flush of saline. While IV administration is preferable, if venous access is not obtained, it is appropriate to give epinephrine via the ETT. In this instances the practitioner, should give a higher dose of Epinephrine: 0.5 to 1 mL/kg of 1:10,000 concentration; (0.05-0.1 mg/kg). Epinephrine can be repeated every five minutes, as needed, while re-evaluating HR every 45 to 60 seconds.
Naloxone
Naloxone (Narcan) is not recommended as an initial response to respiratory distress in neonatal resuscitation. Neonates should be supported on PPV, even in women who have received narcotics less than four hours prior to delivery. However, if respiratory depression continues, naloxone can be considered. In addition, naloxone should be avoided in an infant whose mother has a history of narcotic dependence due to the risk of seizures from withdrawal.
Detection of Hypovolemia
Hypovolemia is detected by measuring arterial blood pressure and by physical examination (i.e., skin color, perfusion, capillary refill time, pulse volume, and extremity temperature).
CVP measurements are useful in detecting hypovolemia and in determining the adequacy of fluid replacement. The venous pressure of normal neonates is 2 to 8 cm H 2 O. If CVP is less than 2 cm H 2 O, hypovolemia should be suspected.
Treatment of Hypovolemia
Treatment of hypovolemia requires expansion of intravascular volume with blood and crystalloid. Albumin may also be used, but evidence of its effectiveness is limited. If it is suspected that the neonate will be hypovolemic at birth, Rh-negative type O packed RBCs should be available in the delivery room before the neonate is born. Crystalloid and blood should be titrated in 10 mL/kg and given slowly over 10 minutes, if hemodynamics allow, to limit the risk of intraventricular hemorrhage.
Occasionally, enormous volumes of blood and fluid are required to raise arterial blood pressure to normal. At times, more than 50% of the blood volume (85 mL/kg in term neonates and 100 mL/kg in preterm neonates) must be replaced, especially when the placenta is transected or abrupted during birth. In most cases, less than 10 to 20 mL/kg of volume restores mean arterial pressure to normal.
Other Causes of Hypotension
Hypoglycemia, hypocalcemia, and hypermagnesemia also cause hypotension in neonates. Hypotension induced by alcohol or magnesium intoxication usually responds to blood volume expansion or dopamine, or to both. Hypermagnesemic neonates generally respond to 100 to 200 mg/kg of calcium gluconate administered over a 5-minute period.
Meconium
Meconium stained amniotic fluid (MSAF) when aspirated into the lungs during delivery or in utero can cause serious lung injury and respiratory distress syndrome (RDS). Most cases of meconium aspiration occur in utero; therefore, endotracheal intubation to suction the airway to remove MSAF should only occur if the neonate is distress: absent or depressed respirations, HR less than 100 bpm, or poor muscle tone. A depressed MSAF stained infant should be intubated as soon as possible following delivery. Suctioning is accomplished through an ETT, and if there is a significant amount of MSAF or the infant remains in extremis, they should be transferred directly to the neonatal ICU.
Color
Essentially all neonates have a blue-tinged cast to their skin at birth. By 60 seconds of age, most of them are entirely pink, except for their hands and feet, which remain blue. If central cyanosis persists beyond 90 seconds of age, asphyxia, low cardiac output, pulmonary edema, methemoglobinemia, polycythemia, congenital heart disease, arrhythmias, and pulmonary disorders (e.g., respiratory distress, airway obstruction, hypoplastic lungs, diaphragmatic hernia) should be considered, especially if the infant remains cyanotic despite oxygen and controlled ventilation. Neonates who are pale at birth are often asphyxiated, hypovolemic, acidotic, or anemic, or they have congenital heart disease. A neonate whose skin is entirely pink within 2 minutes of birth may be intoxicated with alcohol or magnesium or may be alkalotic (pH >7.50). Rubrous neonates are usually polycythemic.
Resuscitation Equipment
Resuscitation beds should allow positioning of the neonate’s head below the level of the lungs to promote drainage of lung fluid and reduce the likelihood of aspirating gastric contents. A servo-controlled infrared heater should be used to maintain the neonate’s temperature between 36°C and 37°C, unless there is evidence of asphyxia. If asphyxia is noted, body temperature should be reduced to 34°C to 35°C for brain protection. A suction device should be available and should allow the suction pressure to be varied; pressures below −100 mm Hg should not be used.
Equipment required for tracheal intubation includes 0 and 00 straight laryngoscope blades; a pencil-type laryngoscope handle; 2.5-, 3.0-, and 3.5-mm ETTs; and a suction catheter that easily fits through each size tube. The ventilation system must permit ventilatory rates of at least 150 breaths/min and make it possible to maintain positive end expiratory pressure (PEEP). One-way valves can stick in the closed position, especially when high gas flow and high RRs are used. The modified Jackson-Rees or Ayres system works well when appropriately trained people use it. Overexpansion of the lungs with large tidal volumes injures the lungs and activates inflammatory processes that may cause chronic lung disease. Gentle inflation of the lung is less injurious to the lung. Airway inflation pressures should be measured continuously during assisted or controlled ventilation in the delivery room, and excessive pressures and tidal volumes should be avoided. As in any critical care situation, patient care should be guided by information. Consequently, blood gas and pH measurements are mandatory, and the results of these tests must be available within 10 minutes of drawing the blood sample. Umbilical arterial catheters are useful for measuring arterial blood pressure and withdrawing blood for blood gas analysis and pHa. They can also be used to infuse emergency fluids. Arterial oxygen saturation (SaO 2 ) can be measured immediately after birth by attaching a pulse oximeter to a hand or foot. Pulse oximeters permit rapid detection of changes in oxygenation and rapid reduction of fraction of inspired oxygen (FiO 2 ). The normal SaO 2 of neonates is usually 87% to 95%, which is associated with a PaO 2 of 55 to 70 mm Hg.
Pediatric Cardiac Arrest and Resuscitation
Pediatric cardiac arrest is not a rare event. At least 16,000 American children (8-20/100,000 children/year) suffer a cardiopulmonary arrest each year. More than half of these cardiac arrests probably occur in-hospital. With advances in resuscitation science and implementation techniques, survival from pediatric cardiac arrest has improved substantially over the past 25 years.
Outcomes from pediatric cardiac arrest have improved significantly over the past 20 years. For example, survival to discharge from pediatric in-hospital cardiac arrest has increased from less than 10% in the 1980s to greater than 25% in the 21st century. Of the pediatric patients that survive to hospital discharge, nearly three quarters will have favorable neurologic function defined by specific pediatric cerebral outcome measures and quality of life indicators. Factors that influence outcome from pediatric cardiac arrest include (1) the pre-existing condition of the child; (2) the environment in which the arrest occurs; (3) the initial ECG rhythm detected; (4) the duration of no-flow time (the time during an arrest without spontaneous circulation or CPR); (5) the quality of the life-supporting therapies provided during the resuscitation; and (6) the quality of the life-supporting therapies during postresuscitation.
Not surprisingly, outcomes after pediatric out-of-hospital arrests are much worse than those after in-hospital arrests. This may be due to the fact that there is a prolonged period of no flow in out-of-hospital arrests, where many of the pediatric cardiac arrests are not witnessed and only 30% of children are provided with bystander CPR. As a result of these factors, less than 10% of cases of pediatric out-of-hospital cardiac arrest (OHCA) survive to hospital discharge, and for those that do survive, severe neurological injury is common. These findings are especially troublesome, given that bystander CPR more than doubles patient survival rates in adults. An exciting prospective, nationwide, population-based cohort study from Japan similarly demonstrates more than doubling of survival rates for children who have OHCA and receive bystander CPR either with conventional CPR (with rescue breathing) or chest compression only CPR compared with no bystander CPR. The same study then further stratifies outcomes for OHCA into “cardiac” and “noncardiac” causes for arrest, and defines the relative value of rescue breathing during CPR by bystanders. Pediatric patients who have OHCA with noncardiac causes and receive bystander conventional CPR (including rescue breathing) had an association with higher frequency of favorable neurologic outcomes at 1 month after arrest compared with compression-only bystander CPR or no bystander CPR. For pediatric arrests defined as “cardiac” in nature, bystander CPR (conventional or compression-only) was associated with a higher rate of favorable neurologic outcomes 1 month after arrest compared with no bystander CPR. Interestingly, the two types of bystander CPR (conventional or compression-only) seemed to be similarly effective for pediatric cardiac arrests with cardiac causes, consistent with animal and adult studies.
Survival outcomes after in-hospital cardiac arrest are higher in the pediatric population compared with adults; 27% of children survive to hospital discharge compared with only 17% of adults. For both children and adults, outcomes are better after arrhythmogenic arrests, ventricular fibrillation (VF)/ventricular tachycardia (VT). Importantly, pediatric in-hospital arrests are less commonly caused by arrhythmias (10% of pediatric arrests vs. 25% of adult arrests), and approximately one-third of children and adults with these arrhythmogenic arrests survive to hospital discharge. Interestingly, the superior pediatric survival rate following in-hospital cardiac arrest reflects a substantially higher survival rate among children with asystole or pulseless electrical activity (PEA) compared with adults (24% vs. 11%). Further investigations have shown that the superior survival rate seen in children is mostly attributable to a much better survival rate among infants and preschool age children compared with older children. Although speculative, the higher survival rates in children may be due to improved coronary and cerebral blood flow (CBF) during CPR because of increased chest compliance in these younger arrest victims, with improved aortic diastolic pressure and venous return. In addition, survival of pediatric patients from an in-hospital cardiac arrest is more likely in hospitals staffed with dedicated pediatric physicians.
Phases of Resuscitation
The four distinct phases of cardiac arrest and CPR interventions are (1) prearrest, (2) no flow (untreated cardiac arrest), (3) low flow (CPR), and (4) postresuscitation/arrest. Interventions to improve outcome of pediatric cardiac arrest should optimize therapies targeted to the time and phase of CPR, as suggested in Table 79.4 .
Phase | Interventions |
---|---|
Prearrest phase (protect) | Optimize patient monitoring and rapid emergency response Recognize and treat respiratory failure or shock to prevent cardiac arrest |
Arrest (no-flow) phase (preserve) | Minimize interval to BLS and ACLS Organize response with clear leadership Minimize interval to defibrillation, when indicated |
Low-flow (CPR) phase (resuscitate) | Push hard, push fast Allow full chest recoil Minimized interruptions in compressions Avoid overventilation Titrate CPR to optimize myocardial blood flow (coronary perfusion pressures and exhaled CO 2 ) Consider adjuncts to improve vital organ perfusion during CPR Consider ECMO if standard CPR/ALS not promptly successful |
Post-resuscitation phase: short-term | Optimize cardiac output and cerebral blood flow Treat arrhythmias, if indicated Avoid hyperglycemia, hyperthermia, hyperventilation Debrief to improve future responses to emergencies |
Postresuscitation phase: long-term rehabilitation (regenerate) | Early intervention with occupational and physical therapy Bioengineering and technology interface Possible future role for stem cell transplantation |
Prearrest
The prearrest phase refers to any relevant preexisting conditions of the child (e.g., neurologic, cardiac, respiratory, or metabolic problems) and precipitating events (e.g., respiratory failure or shock), uncoupling metabolic delivery and metabolic demand. Pediatric patients who suffer an in-hospital cardiac arrest often have changes in their physiological status in the hours leading up to their arrest event. Therefore, interventions during the prearrest phase focus on preventing the cardiac arrest, with special attention to early recognition and targeted treatment of respiratory failure and shock. Early recognition plays a key role in identifying a prearrest state in children, who unlike adults may be able to mount a prolonged physiologic response to a worsening clinical picture. Medical emergency teams (METs; also known as rapid response teams ) are in-hospital emergency teams designed specifically for this purpose. Front-line providers, and even parents, are encouraged to initiate evaluation by METs based on physiologic protocol driven parameters or even intuition. Patients are assessed by the METs, and those at high risk of clinical decompensation are transferred to a pediatric ICU if necessary, with the goal to prevent progression to full cardiac arrest or to decrease the response time to initiation of advanced life support, thereby limiting the no-flow state. Implementation of METs decreases the frequency of cardiac arrests compared with retrospective control periods before MET initiation. While early recognition protocols cannot identify all children at risk for cardiac arrest, it seems reasonable to assume that transferring critically ill children to an ICU early in their disease process for better monitoring and more aggressive interventions can improve resuscitative care and clinical outcomes. The caveat is that prearrest states must be identified to initiate monitoring and interventions that may inhibit the progression to an arrest. While a significant amount of research dollars and resources are spent on the other phases of cardiac arrest, particular focus on the prearrest state may yield the greatest improvement in survival and neurologic outcomes.
No Flow/Low Flow
Airway-Breathing-Circulation or Circulation-Airway-Breathing
For OHCA victims, “compression-only” CPR has been associated with improved outcomes. This is now the recommended modality for emergency medical service dispatcher instructing bystander CPR. In a recent Japanese study, children with OHCA due to a primary cardiac etiology displayed an equivalent survival rate between compression-only CPR and classic CPR with rescue breaths. However, only 29% of patients had a cardiac cause of OHCA. Those with noncardiac etiology in the overall cohort had a significantly worse survival rate with compression-only CPR, as compared with classic CPR with rescue breaths. Additionally, in another nationwide Japanese OHCA registry study, compression-only CPR was superior to no bystander CPR at all but not to conventional CPR. In a recent American OHCA registry study, children who received conventional bystander CPR with chest compressions and rescue breaths had improved rates of overall survival and survival with favorable outcomes as compared with those who did not receive CPR, whereas those receiving compression-only CPR did not fare any better than children not receiving CPR. Thus compression-only CPR is not recommended for children in either the inpatient or out-of-hospital setting, except in situations in which “rescuers are unwilling or unable to deliver breaths.”
Regardless, the prioritization of initial interventions during CPR has shifted from airway-breathing-circulation (“A-B-C”) to circulation-airway-breathing (“C-A-B”) in order to prevent harmful delays in the initiation of chest compressions and due to the relative complexity of the tasks involved in providing assisted ventilation. This is endorsed by both the 2010 and 2015 AHA BLS Guidelines. However, a 2015 International Liaison Committee on Resuscitation consensus statement identified a paucity of pediatric-specific evidence to support this recommendation. In our opinion, the approach is physiologically sound, especially given the association of delayed chest compression initiation with poor outcomes. With that said, the pediatric provider must consider the predominance of asphyxia and hypoxemia as precursors to cardiac arrest. This is especially true in the ICU and operating room, where personnel and other resources frequently allow for simultaneous circulatory support with high-quality chest compressions as well as the provision of assisted ventilations by experienced personnel.
In order to improve outcomes from pediatric cardiac arrest, it is imperative to shorten the no-flow phase of untreated cardiac arrest. To that end, it is important to monitor high-risk patients to allow early recognition of the cardiac arrest and prompt initiation of basic and advanced life support. Effective CPR optimizes coronary perfusion pressure (by elevating aortic diastolic pressure relative to RAP) and cardiac output to critical organs to support vital organ viability (by elevating mean aortic pressure) during the low flow phase. Important tenets of basic life support are push hard, push fast, allow full chest recoil between compressions, and minimize interruptions of chest compression . The myocardium receives blood flow from the aortic root, mainly during diastole, via the coronary arteries. When the heart arrests and no blood flows through the aorta, coronary blood flow ceases. However, during chest compressions, aortic pressure rises at the same time as RAP and with the subsequent decompression phase of chest compressions, the RAP falls faster and lower than the aortic pressure, which generates a pressure gradient that perfuses the heart with oxygenated blood. Therefore, full elastic recoil (release) is critical to create a pressure difference between the aortic root and the right atrium. A CPP below 15 mm Hg during CPR is a poor prognostic factor for ROSC. Achieving optimal coronary perfusion pressure, exhaled carbon dioxide concentration, and cardiac output during the low flow phase of CPR is consistently associated with an improved chance for return of spontaneous circulation (ROSC) and improved short- and long-term outcome in mature animal and human studies. There is a critical need for research evaluating goal directed CPR, both in immature animal models and pediatric patients. Other measures essential for truncating the no-flow phase during VF and pulseless VT are rapid detection and prompt defibrillation. Clearly, CPR alone is inadequate for successful resuscitation from these arrhythmias. For cardiac arrests resulting from asphyxia and/or ischemia, provision of adequate myocardial perfusion and myocardial oxygen delivery are the critical elements for ROSC.
Postarrest/Resuscitation
The postarrest/resuscitation phase includes coordinated, skilled management of the immediate post-resuscitation stage, the next few hours to days, and long-term rehabilitation. The immediate post-resuscitation stage is a high-risk period for ventricular arrhythmias and other reperfusion injuries. Goals of interventions implemented during the immediate post-resuscitation stage and the next few days include adequate tissue oxygen delivery, treatment of post-resuscitation myocardial dysfunction, and minimizing post-resuscitation tissue injury (e.g., preventing post-resuscitation hyperthermia and hypoglycemia; and, perhaps initiating post-resuscitation therapeutic hypothermia, preventing hyperglycemia and avoiding hyperoxia). This post-arrest/resuscitation phase may have the greatest potential for innovative advances in the understanding of cell injury (excitotoxicity, oxidative stress, metabolic stress) and cell death (apoptosis and necrosis), ultimately leading to novel molecular-targeted interventions. The rehabilitation stage concentrates on salvage of injured cells, and support for reengineering of reflex and voluntary communications of these cell and organ systems to improve long-term functional outcome.
The specific phase of resuscitation dictates the focus of care. Interventions that improve outcome during one phase may be deleterious during another. For instance, intense vasoconstriction during the low flow phase of cardiac arrest improves coronary perfusion pressure and the probability of ROSC. The same intense vasoconstriction during the post-resuscitation phase increases left ventricular afterload and may worsen myocardial strain and dysfunction. Current understanding of the physiology of cardiac arrest and recovery allows us to only crudely manipulate blood pressure, oxygen delivery and consumption, body temperature, and other physiologic parameters in our attempts to optimize outcome. Future strategies likely will take advantage of increasing knowledge of cellular injury, thrombosis, reperfusion, mediator cascades, cellular markers of injury and recovery, and transplantation technology, including stem cells.
Interventions During the Cardiac Arrest (No Flow) and Cardiopulmonary Resuscitation (Low Flow)
Airway and Breathing
During CPR, cardiac output and pulmonary blood flow are ∼10% to 25% of that during normal sinus rhythm; therefore, a lower minute ventilation is necessary for adequate gas exchange from the blood traversing the pulmonary circulation. Animal and adult data indicate that overventilation (“overventilation” from exuberant rescue breathing) during CPR is common and can substantially compromise venous return and subsequently cardiac output. These detrimental hemodynamic effects are compounded when one considers the effect of interruptions in CPR to provide airway management and rescue breathing and may contribute to worse survival outcomes. While overventilation is problematic, in light of the fact that most pediatric arrests are asphyxial in nature, immediate initiation of adequate ventilation is still important. The difference between arrhythmogenic and asphyxial arrests lies in the physiology. In animal models of sudden VF cardiac arrest, acceptable PaO 2 and PaCO 2 persist for 4 to 8 minutes during chest compressions without rescue breathing. This is in part because aortic oxygen and carbon dioxide concentrations at the onset of the arrest do not vary much from the prearrest state with no blood flow and minimal aortic oxygen consumption. The lungs act as a reservoir of oxygen during the low-flow state of CPR; therefore, adequate oxygenation and ventilation can continue without rescue breathing. Several retrospective studies of witnessed VF cardiac arrest in adults have also shown that outcomes are similar after bystander-initiated CPR with either chest compressions alone or chest compressions plus rescue breathing. However, during asphyxial arrest, peripheral and pulmonary blood flow continues during the prearrest, state resulting in significant arterial and venous oxygen desaturation, elevated lactate levels, and depletion of the pulmonary oxygen reserve. Therefore, at the onset of CPR, there is substantial arterial hypoxemia and resulting acidemia. In this circumstance, rescue breathing with controlled ventilation can be a life-saving maneuver. In contrast, the adverse hemodynamic effects from overventilation during CPR combined with possible interruptions in chest compressions to open the airway and deliver rescue breathing are a lethal combination in certain circumstances such as VT/VF arrests. In short, the resuscitation technique should be titrated to the physiology of the patient to optimize patient outcome.
Circulation: Optimizing Blood Flow During Low Flow Cardiopulmonary Resuscitation: Push Hard, Push Fast
When the heart arrests, no blood flows to the aorta and coronary blood flow ceases immediately. At that point, provision of high quality CPR (PUSH HARD, PUSH FAST) is vital to reestablish coronary flow. The goal during CPR is to maximize the myocardial perfusion pressure (MPP). Related by the following equation: MPP = aortic diastolic blood pressure (AoDP) minus RAP, myocardial blood flow improves as the gradient between AoDP and RAP increases. During downward compression phase, aortic pressure rises at the same time as RAP with little change in the MPP. However, during the decompression phase of chest compressions, the RAP falls faster and lower than the aortic pressure, which generates a pressure gradient perfusing the heart with oxygenated blood during this artificial period of “diastole.” Several animal and human studies have demonstrated, in both VT/VF and asphyxial models, the importance of establishing MPP as a predictor for short-term survival outcome (ROSC). Because there is no flow without chest compressions, it is important to minimize interruptions in chest compressions. To allow good venous return in the decompression phase of external cardiac massage, it is also important to allow full chest recoil and to avoid overventilation (preventing adequate venous return because of increased intrathoracic pressure).
Based on the provided equation, MPP can be improved by strategies that increase the pressure gradient between the aorta and the right atrium. As an example, the inspiratory impedance threshold device (ITD) is a small, disposable valve that can be connected directly to the tracheal tube or face mask to augment negative intrathoracic pressure during the inspiratory phase of spontaneous breathing and the decompression phase of CPR by impeding airflow into the lungs. Application in animal and adult human trials of CPR has established the ability of the ITD to improve vital organ perfusion pressures and myocardial blood flow ; however, in the only randomized trial during adult CPR, mortality benefit was limited to the subgroup of patients with PEA. Additional evidence that augmentation of negative intrathoracic pressure can improve perfusion pressures during CPR comes from the active compression-decompression device (ACD). The ACD is a handheld device that is fixed to the anterior chest of the victim by means of suction similar to a household plunger that can be used to apply active decompression forces during the release phase, thereby creating a vacuum within the thorax. By actively pulling during the decompression phase, blood is drawn back into the heart by the negative pressure. Animal and adult studies have demonstrated that the combination of ACD with ITD act in concert to further improve perfusion pressures during CPR compared with ACD alone. In the end, while novel interventions such as the ITD and ACD are promising adjuncts to improve blood flow during CPR, the basic tenants of PUSH HARD, PUSH FAST, ALLOW FULL CHEST WALL RELEASE, MINIMIZE INTERRUPTIONS, and DON’T OVERVENTILATE are still the dominate factors to improve blood flow during CPR and chance of survival.
Chest Compression Depth
The pediatric chest compression depth recommendation of at least one-third anterior-posterior chest depth (approximately 4 cm in infants and 5 cm in children) is based largely upon expert clinical consensus, using data extrapolated from animal, adult, and limited pediatric data. In a small study of six infants, chest compressions targeted to one half anterior-posterior chest depth resulted in improved systolic blood pressures, compared with those targeted at one-third anterior-posterior chest depth. While only a small series with qualitatively estimated chest compression depths, this is the first study to collect actual data from children supporting the existing chest compression depth guidelines. On the contrary, two recent studies using computer automated tomography (CT) suggest that depth recommendations based on a relative (%) anterior-posterior chest compression depth are deeper than that recommended for adults, and that a depth of one half anterior-posterior chest depth will result in direct compression to the point of fully emptying the heart and requisite shifting of heart because of inadequate AP diameter reserve in most children. Future studies that collect data from actual children and that associate quantitatively measured chest compression depths with short- and long-term clinical outcomes (arterial blood pressure, end tidal carbon dioxide, ROSC, survival) are needed.
Compression/Ventilation Ratios
The amount of ventilation provided during CPR should match, but not exceed, perfusion and should be titrated to the amount of circulation during the specific phase of resuscitation, as well as the metabolic demand of the tissues. Therefore, during the low flow state of CPR when the amount of cardiac output is roughly 10% to 25% of normal, less ventilation is needed. However, the best ratio of compressions to ventilations in pediatric patients is largely unknown and depends on many factors, including the compression rate, the tidal volume, the blood flow generated by compressions, and the time that compressions are interrupted to perform ventilation. Recent evidence demonstrates that a compression/ventilation ratio of 15:2 delivers the same minute ventilation and increases the number of delivered chest compressions by 48% compared with CPR at a compression/ventilation ratio of 5:1 in a simulated pediatric arrest model. This is important because when chest compressions cease, the aortic pressure rapidly decreases and coronary perfusion pressure falls precipitously, thereby decreasing myocardial oxygen delivery. Increasing the ratio of compressions to ventilations minimizes these interruptions, thus increasing coronary blood flow. The benefits of PPV (increased arterial content of oxygen and carbon dioxide elimination) must be balanced against the adverse consequence of decreased circulation. These findings are in part the reason the AHA now recommends a pediatric compression/ventilation ratio of 15:2 .
Duty Cycle
In a model of human adult cardiac arrest, cardiac output and coronary blood flow are optimized when chest compressions last for 30% of the total cycle time (approximately 1:2 ratio of time in compression to time in relaxation). As the duration of CPR increases, the optimal duty cycle may increase to 50%. In a juvenile swine model, a relaxation period of 250 to 300 milliseconds (duty cycle of 40%-50% at a compression rate of 120/min) correlates with improved cerebral perfusion pressures (CPPs) compared with shorter duty cycles of 30%.
Circumferential Versus Focal Sternal Compressions
In adult and animal models of cardiac arrest, circumferential (vest) CPR has been demonstrated to dramatically improve CPR hemodynamics. In smaller infants, it is often possible to encircle the chest with both hands and depress the sternum with the thumbs, while compressing the thorax circumferentially (thoracic squeeze). In an infant animal model of CPR, this “two-thumb” method of compression with thoracic squeeze resulted in higher systolic and diastolic blood pressures and a higher pulse pressure than traditional two-finger compression of the sternum. Although not rigorously studied, our clinical experience indicates that it is very difficult to attain adequate chest compression force and adequate aortic pressures with the two-finger technique, so we fully support the AHA Guidelines for health care providers to perform CPR on infants with the two-thumb-encircling hands technique.
Open-Chest Cardiopulmonary Resuscitation
In animal models, high quality standard, closed-chest CPR generates myocardial blood flow that is greater than 50% of normal, CBF that is approximately 50% of normal, and cardiac output ∼10% to 25% of normal. By contrast, open-chest CPR can generate myocardial and CBF that approaches normal. Although open-chest massage improves coronary perfusion pressure and increases the chance of successful defibrillation in animals and humans, performing a thoracotomy to allow open-chest CPR is impractical in many situations. A retrospective review of 27 cases of CPR following pediatric blunt trauma (15 with open-chest CPR and 12 with closed-chest CPR) demonstrated that open-chest CPR increased hospital cost without altering rates of ROSC or survival to discharge. However, survival in both groups was 0%, indicating that the population may have been too severely injured or too late in the process to benefit from this aggressive therapy. Open-chest CPR is often provided to children after open-heart cardiac surgery and sternotomy. Earlier institution of open-chest CPR may warrant reconsideration in selected special resuscitation circumstances.
Medications Used to Treat Cardiac Arrest
While animal studies have indicated that epinephrine can improve initial resuscitation success after both asphyxial and VF cardiac arrests, there are no prospective studies to support the use of epinephrine or any other medication to improve survival outcome from pediatric cardiac arrest. A variety of medications are used during pediatric resuscitation attempts, including vasopressors (epinephrine and vasopressin), antiarrhythmics (amiodarone and lidocaine), and other drugs such as calcium chloride and sodium bicarbonate. Each will be discussed separately as follows.
Vasopressors
Epinephrine (adrenaline) is an endogenous catecholamine with potent α- and β-adrenergic stimulating properties. The α-adrenergic action (vasoconstriction) increases systemic and PVR. The resultant higher aortic diastolic blood pressure improves coronary perfusion pressure and myocardial blood flow, even though it reduces global cardiac output during CPR; as noted previously, adequacy of myocardial blood flow is a critical determinant of ROSC. Epinephrine also increases CBF during good quality CPR because peripheral vasoconstriction directs a greater proportion of flow to the cerebral circulation. However, recent evidence suggests that epinephrine can decrease local cerebral microcirculatory blood flow at a time when global cerebral flow is increased. The β-adrenergic effect increases myocardial contractility and HR, and relaxes smooth muscle in the skeletal muscle vascular bed and bronchi; however, the β-adrenergic effects are not observed in the peripheral vascular beds secondary to the high dose used in cardiac arrest. Epinephrine also increases the vigor and intensity of VF, increasing the likelihood of successful defibrillation. High-dose epinephrine (0.05-0.2 mg/kg) improves myocardial and CBF during CPR more than standard-dose epinephrine (0.01-0.02 mg/kg) in animal models of cardiac arrest and may increase the incidence of initial ROSC. However, prospective and retrospective studies have indicated that the use of high-dose epinephrine in adults or children does not improve survival and may be associated with worse neurologic outcome. A randomized, blinded, controlled trial of rescue high-dose epinephrine versus standard-dose epinephrine after failed initial standard dose epinephrine in pediatric in-hospital cardiac arrest demonstrated a worse 24-hour survival in the high-dose epinephrine group (1 of 27 survivors vs. 6 of 23 survivors, P < .05). Based on these clinical studies, high-dose epinephrine cannot be recommended routinely for either initial or rescue therapy. Importantly, these studies indicate that high-dose epinephrine can worsen a patient’s post-resuscitation hemodynamic condition and likelihood of survival.
Vasopressin is a long-acting endogenous hormone that acts at specific receptors to mediate systemic vasoconstriction (V 1 receptor) and reabsorption of water in the renal tubule (V 2 receptor). Vasoconstrictive properties are most intense in the skeletal muscle and skin vascular beds. Unlike epinephrine, vasopressin is not a pulmonary vasoconstrictor. In experimental models of cardiac arrest, vasopressin increases blood flow to the heart and brain and improves long-term survival compared with epinephrine. However, vasopressin can decrease splanchnic blood flow during and following CPR and can increase afterload in the post-resuscitation period placing further strain on the left ventricle. Adult randomized, controlled trials suggest that outcomes are similar after use of vasopressin or epinephrine during CPR. During pediatric arrest, a case series of four children who received vasopressin during six prolonged cardiac arrest events suggested that the use of bolus vasopressin may result in ROSC when standard medications have failed. However, a more recent retrospective study of 1293 consecutive pediatric arrests from the National Registry of CPR (NPCRP) found that vasopressin use, while infrequent (administered in only 5% of events), was associated with a lower likelihood of ROSC. Therefore, it is unlikely that vasopressin will replace epinephrine as a first-line agent in pediatric cardiac arrest. However, the available data suggest that its use in conjunction with epinephrine may deserve further investigation, especially in prolonged arrest unresponsive to initial epinephrine resuscitation.
Antiarrhythmic Medications
Calcium
Calcium is used frequently in cases of pediatric cardiac arrest, despite the lack of evidence for efficacy. In the absence of a documented clinical indication (i.e., hypocalcemia, calcium channel blocker overdose, hypermagnesemia, or hyperkalemia), administration of calcium does not improve outcomes from cardiac arrest. To the contrary, three pediatric studies have suggested a potential for harm, as routine calcium administration was associated with decreased survival rates and / or worse neurological outcomes. Despite limited clinical data to support the use of calcium during CPR, it is reasonable to consider calcium administration during CPR for cardiac arrest patients at high risk of hypocalcemia (e.g., renal failure, shock associated with massive transfusion, etc.).
Buffer Solutions
There are no randomized controlled studies in children examining the use of sodium bicarbonate for management of pediatric cardiac arrest. Two randomized controlled studies have examined the value of sodium bicarbonate in the management of adult cardiac arrest and in neonates with respiratory arrest in the delivery room. Neither study was associated with improved survival. In fact, one multicenter retrospective in-hospital pediatric study found that sodium bicarbonate administered during cardiac arrest was associated with decreased survival, even after controlling for age, gender and first documented cardiac rhythm. Therefore, during pediatric cardiac arrest resuscitation, the routine use of sodium bicarbonate is not recommended. Clinical trials involving critically ill adults with severe metabolic acidosis do not demonstrate a beneficial effect of sodium bicarbonate on hemodynamics despite correction of acidosis. This is somewhat surprising in light of data that severe acidosis may depress the action of catecholamines and worsen myocardial function. Nevertheless, the common use of sodium bicarbonate during CPR is not supported by clinical data. Pediatric patients with implanted cardiac pacemakers may have an increased threshold for myocardial electrical stimulation when acidotic ; therefore, administration of bicarbonate or another buffer is appropriate for management of severe documented acidosis in these children. Administration of sodium bicarbonate also is indicated in the patient with a tricyclic antidepressant overdose, hyperkalemia, hypermagnesemia, or sodium channel blocker poisoning. The buffering action of bicarbonate occurs when a hydrogen cation and a bicarbonate anion combine to form carbon dioxide and water. Carbon dioxide must be cleared through adequate minute ventilation; thus, if ventilation is impaired during sodium bicarbonate administration, carbon dioxide buildup may negate the buffering effect of bicarbonate. Because carbon dioxide readily penetrates cell membranes, intracellular acidosis may paradoxically increase after sodium bicarbonate administration without adequate ventilation. Therefore, bicarbonate should not be used for management of respiratory acidosis.
Unlike sodium bicarbonate, tromethamine (THAM) buffers excess protons without generating carbon dioxide; in fact, carbon dioxide is consumed following THAM administration. In a patient with impaired minute ventilation, tromethamine may be preferable when buffering is necessary to mitigate severe acidosis. Tromethamine undergoes renal elimination, and renal insufficiency may be a relative contraindication to its use. Carbicarb, an equimolar combination of sodium bicarbonate and sodium carbonate, is another buffering solution that generates less carbon dioxide than sodium bicarbonate. In a canine model of cardiac arrest comparing animals given normal saline, sodium bicarbonate, THAM, or Carbicarb, the animals given any buffer solution had a higher rate of ROSC than the animals given normal saline. In the animals given sodium bicarbonate or Carbicarb, the interval to ROSC was significantly shorter than in animals given normal saline. However, at the end of the 6-hour study period, all resuscitated animals were in a deep coma, so no inferences regarding meaningful survival can be drawn. It is premature to recommend either THAM or Carbicarb during CPR at this time.
Post-Resuscitation Interventions
Temperature Management
Two seminal articles have established that induced hypothermia (32°C-34°C) could improve outcome for comatose adults after resuscitation from VF cardiac arrest. In both randomized, controlled trials, the inclusion criteria were patients older than 18 years who were persistently comatose after successful resuscitation from nontraumatic VF. However, in a recent randomized control trial with unconscious adult survivors of OHCA, a targeted temperature of 33°C did not confer a benefit as compared with the targeted temperature of 36°C. Interpretation and extrapolation of these studies to children is difficult; however, fever within the first 48 hours following cardiac arrest, brain trauma, stroke, and ischemia is associated with poor neurologic outcome. Emerging neonatal trials of selective brain cooling and systemic cooling show promise in neonatal HIE, suggesting that induced hypothermia may improve outcomes. The efficacy of therapeutic hypothermia following pediatric cardiac arrest is being evaluated in a randomized controlled trial ( clinicaltrials.gov identifier NCT00880087); THAPCA: Therapeutic Hypothermia After Pediatric Cardiac Arrest ( www.thapca.org ). At a minimum, it is advisable to avoid hyperthermia in children following CPR. Using an approach of “therapeutic normothermia” with scheduled administration of antipyretic medications and the use of external cooling devices, while monitoring core temperature, may be necessary to prevent hyperthermia in this population. Notably, preventing hyperthermia is not easy. Many children become hyperthermic post-arrest despite the intent to prevent hypothermia.
Glucose Control
Both hyperglycemia and hypoglycemia following cardiac arrest is associated with worse neurologic outcome. While it seems intuitive that hypoglycemia would be associated with worse neurologic outcome, whether hyperglycemia per se is harmful or is simply a marker of the severity of the stress hormone response from prolonged ischemia is not clear. A recent randomized control trial suggests that tight glycemic control in critically ill children had no effect on major clinical outcomes, but was associated with a higher incidence of hypoglycemia. In summary, there is insufficient evidence to formulate a strong recommendation on the management of hyperglycemia in children with ROSC following cardiac arrest. If hyperglycemia is treated following ROSC in pediatric patients, blood glucose concentrations should be carefully monitored to avoid hypoglycemia.
Blood Pressure Management
A patient with ROSC may have substantial variability in blood pressure following cardiac arrest. Postarrest/resuscitation myocardial dysfunction is very common and is often associated with hypotension (discussed later). In addition, hypertension may occur, especially if the patient receives vasoactive infusions for postarrest myocardial dysfunction. Optimization of blood pressure postarrest is critical to maintain adequate perfusion pressure to vital organs that may have already been injured from the “no flow” and “low blood flow” states during initial cardiac arrest and CPR. Cerebral blood flow in healthy patients is tightly controlled over a wide range of mean arterial blood pressure via cerebral neurovascular bundle (autoregulation); however, adults resuscitated from cardiac arrest have demonstrated impaired autoregulation of CBF, and this may also be the case in children. Dysautoregulation of the cerebral neurovascular bundle following cardiac arrest may limit the brain’s ability to regulate excessive blood flow and microvascular perfusion pressure, thereby leading to reperfusion injury during systemic hypertension. However, in animal models, brief induced hypertension following resuscitation results in improved neurologic outcome compared with normotensive reperfusion. Conversely, systemic hypotension may perpetuate neurologic metabolic crisis following ischemic injury by uncoupling bioenergetic demand and delivery. Therefore, a practical approach to blood pressure management following cardiac arrest is to attempt to minimize blood pressure variability in this high-risk period following resuscitation.
Post-resuscitation Myocardial Dysfunction
Postarrest myocardial stunning and arterial hypotension occur commonly after successful resuscitation in both animals and humans. Animal studies demonstrate that postarrest myocardial stunning is a global phenomenon with biventricular systolic and diastolic dysfunction. Postarrest myocardial stunning is pathophysiologically and physiologically similar to sepsis-related myocardial dysfunction and postcardiopulmonary bypass myocardial dysfunction, including increases in inflammatory mediators and NO production. Because cardiac function is essential to reperfusion following cardiac arrest, management of postarrest myocardial dysfunction may be important to improving survival. The classes of agents used to maintain circulatory function (i.e., inotropes, vasopressors, and vasodilators) must be carefully titrated during the post-resuscitation phase to the patient’s cardiovascular physiology. Although the optimal management of post–cardiac arrest hypotension and myocardial dysfunction has not been established, data suggest that aggressive hemodynamic support may improve outcomes. Controlled trials in animal models have shown that dobutamine, milrinone, levosimendan can effectively ameliorate post–cardiac arrest myocardial dysfunction. In clinical observational studies, fluid resuscitation has been provided for patients with hypotension and concomitant low central venous pressure, and various vasoactive infusions, including epinephrine, dobutamine, and dopamine, have been used to treat the myocardial dysfunction syndrome. In the end, optimal use of these agents involves close goal-directed titration, and the use of invasive hemodynamic monitoring may be appropriate. General critical care principles suggest that appropriate therapeutic goals are adequate blood pressures and adequate oxygen delivery. However, the definition of “adequate” is elusive. Reasonable interventions for vasodilatory shock with low central venous pressure include fluid resuscitation and vasoactive infusions. Appropriate considerations for left ventricular myocardial dysfunction include euvolemia, inotropic infusions, and afterload reduction.
Neuromonitoring
Continuous neuromonitoring and goal-directed intervention following cardiac arrest is an exciting frontier with great promise in improving neurologic outcomes post–cardiac arrest. Continuous electroencephalogram (cEEG) monitoring is an increasingly instituted modality for neuromonitoring of critically ill patients, especially to diagnose nonconvulsive seizures (NCS) and seizures in patient’s receiving muscle relaxants. cEEG monitoring is noninvasive, performed at the bedside, and permits continuous assessment of cortical function. Interpretation of continuous electroencephalogram (EEG) is usually performed by a neurologist from a remote location, and not bedside critical care physicians. However, advances in quantitative EEG tools may allow bedside caregivers to identify important electrographic events, such as seizures or abrupt background changes, to potentially permit real-time analysis and intervention. In a prospective study of cEEG in children, NCS were detected in 39% (12 of 31) children following cardiac arrest. In a partially overlapping cohort of 19 children, NCS were common in children undergoing therapeutic hypothermia after cardiac arrest. NCS seems to be a common occurrence following cardiac arrests in children. Although the relationship of NCS to worse outcomes has not been established in pediatric patients following cardiac arrest, it has been associated with worse outcomes among critically ill adults and neonates. We believe that cEEG should be considered for children post–cardiac arrest and that patients with NCS (especially status epilepticus with NCS) should be treated with anticonvulsant medication. Further study is warranted to better establish frequency of NCS and potential benefit in outcomes with anticonvulsant therapy.
Oxidative injury may be greatest in the early phases of post-resuscitation therapy following cardiac arrest. Interestingly, the use of 100% oxygen (compared with room air) during and immediately following resuscitation in animal models may potentiate oxidative injury to key mitochondrial enzymes (pyruvate dehydrogenase or manganese superoxide) or mitochondrial lipids (cardiolipin), and is associated with worse neurologic outcomes. Experimental protocols in large animals using peripheral pulse-oximetry to titrate oxygenation in the post-resuscitation phase can reduce post-resuscitation hyperoxia and significantly improve neuropathology and neurobehavioral outcomes. Consistent with these experimental findings, arterial hyperoxia (PaO 2 ≥ 300 mm Hg) was independently associated with in-hospital mortality compared with either hypoxia or normoxia in an observational study among critically ill adult patients admitted to the ICU within 24 hours of a cardiac arrest. We believe it is prudent to titrate oxygenation during and following pediatric cardiac arrest. Although the optimal SpO 2 is not known, we recommend titration of FiO 2 to the lowest amount necessary to assure SpO 2 >94%. Perhaps the future of post-arrest care will include more aggressive neurocritical care monitoring, such as near infrared spectroscopy, cerebral microdialysis, PbtO 2 , CBF, and even bedside analysis of mitochondrial dysfunction.
Quality of Cardiopulmonary Resuscitation
Despite evidence-based guidelines, extensive provider training, and provider credentialing in resuscitation medicine, the quality of CPR is typically poor. CPR guidelines recommend target values for selected CPR parameters related to rate and depth of chest compressions and ventilations, avoidance of CPR-free intervals, and complete release of sternal pressure between compressions. Slow compression rates, inadequate depth of compression, and substantial pauses are the norm. An approach to Push Hard, Push Fast, Minimize Interruptions, Allow Full Chest Recoil, and Don’t Overventilate can markedly improve myocardial, cerebral, and systemic perfusion, and will likely improve outcomes. Quality of post-resuscitative management has also been demonstrated to be critically important to improve resuscitation survival outcomes. Measuring the quality of CPR and avoiding overventilation during cardiac arrest resuscitation have recently been reemphasized by consensus of the International Liaison Committee on Resuscitation and the AHA. Although the correct amount, timing, intensity, and duration of ventilation that is required during CPR is controversial, there is no controversy that measurement and titration of the amount of ventilation to the amount of blood perfusion are desirable. Thus additional technology that is safe, accurate, and practical would improve detection and feedback of the “quality of CPR.”
Recent technology has been developed that monitors quality of CPR by force sensors and accelerometers, and can provide verbal feedback to the CPR administrator regarding the frequency and depth of chest compressions and the volume of ventilations. Recent pediatric data illustrates that intensive training and real-time corrective feedback can help chest compression quality approach age-specific AHA CPR guideline targets. Moreover, improvements in post-resuscitation care can improve resuscitation survival outcomes.
Extracorporeal Membrane Oxygenation-Cardiopulmonary Resuscitation
The use of extracorporeal membrane life-saving (ECLS) devices as a rescue therapy for refractory cardiac arrest (ECPR) is an exciting topic in resuscitation science. In children with medical or surgical cardiac diseases, ECPR has been shown to improve survival to hospital discharge and can be effective after even greater than 50 minutes of CPR. However, at this time, observational data has not consistently demonstrated a survival benefit of ECPR compared with conventional CPR across broad populations. Children with primary cardiac disease may have a survival advantage, due to these disease processes being amenable to a bridge with ECLS—whether to recovery, surgery, or transplantation. There may be an underlying advantage for these patients as well, stemming from predominantly single-organ failure compared with patients with noncardiac etiologies of cardiac arrest, allowing for a greater chance of full recovery after resuscitation. Importantly, in these observational studies, ECPR is used as a rescue therapy in patients who likely would have died with continued conventional resuscitation efforts. In fact, in a GWTG-R study that looked at both cardiac and noncardiac patients with greater than 10 minutes of CPR, those who received ECPR had improved survival and favorable neurologic outcome at discharge. A lack of survival advantage, even when controlling for confounding factors, is flawed by the nature of these studies. In the absence of randomized controlled trials that specifically compare early initiation of ECPR and conventional CPR, it is probably reasonable to consider ECPR as a rescue therapy in patients with potentially reversible underlying disease processes. However, as noted in PALS guidelines, any reasonable chance of success requires a setting with “existing ECMO protocols, expertise, and equipment,” and dedicated teams that train for efficient cannulation under difficult circumstances. Therefore, timely, quality E-CPR may be an exciting adjuvant to conventional CPR for pediatric patients. Future frontiers will define patient populations and optimize the clinical approach to extracorporeal support; however, clinicians providing CPR should consider E-CPR early in the course of a resuscitation not responding to conventional CPR. Perhaps after failure to attain ROSC within 5 minutes, clinicians should ask themselves: (1) does the patient have a potentially reversible process, (2) would ECMO be a “bridge” to a potentially good outcome, and (3) do we have the personnel and resources to provide EMCO promptly? If the answer to all three are “yes,” prompt implementation of E-CPR should be considered. We believe that patients arriving with a witnessed arrest with immediate initiation of CPR and evidence of quality CPR should be considered E-CPR candidates.
Ventricular Fibrillation and Ventricular Tachycardia in Children
Pediatric VF, or VT, has been an underappreciated pediatric problem. Recent studies indicate that VF and VT (i.e., shockable rhythms) occur in 27% of in-hospital cardiac arrests at some time during the resuscitation. In a population of pediatric cardiac ICU patients, as many as 41% of arrests were associated with VF or VT. According to the National Registry of Cardiopulmonary Resuscitation (NRCPR) database, 10% of children with in-hospital cardiac arrest had an initial rhythm of VF/VT. In all, 27% of the children had VF/VT at some time during the resuscitation. The incidence of VF varies by setting and age. In special circumstances, such as tricyclic antidepressant overdose, cardiomyopathy, post–cardiac surgery, and prolonged QT syndromes, VF and pulseless VT are more likely. The treatment of choice for short-duration VF is prompt defibrillation. In general, the mortality rate increases by 7% to 10% per minute of delay to defibrillation. Because VF must be considered before defibrillation can be provided, early determination of the rhythm by ECG is critical. An attitude that VF is rare in children can be a self-fulfilling prophecy with a uniformly fatal outcome. The recommended defibrillation dose is 2 J/kg, but the data supporting this recommendation are not optimal and are based on old monophasic defibrillators. In the mid-1970s, authoritative sources recommended starting doses of 60 to 200 J for all children. Because of concerns for myocardial damage and animal data suggesting that shock doses ranging from 0.5 to 1 J/kg were adequate for defibrillation in a variety of species, Gutgesell et al. evaluated the efficacy of their strategy to defibrillate with 2 J/kg monophasic shocks. Seventy-one transthoracic defibrillations in 27 children were evaluated. Shocks within 10 J of 2 J/kg resulted in successful defibrillation (i.e., termination of fibrillation) in 91% of defibrillation attempts. More recent data demonstrate that an initial shock dose of 2 J/kg terminates fibrillation in less than 60% of children, suggesting that a higher dose may be needed. Interestingly, retrospective observational NRCPR data demonstrate that higher initial doses of 4 J/kg were associated with worse short-term survival (i.e., immediate survival of the cardiac arrest event with a spontaneous rhythm). Despite 5 decades of clinical experience with pediatric defibrillation, the optimal dose remains unknown.
Antiarrhythmic Medications: Lidocaine and Amiodarone
Administration of antiarrhythmic medications should never delay administration of shocks to a patient with VF. However, after an unsuccessful attempt at electrical defibrillation, medications to increase the effectiveness of defibrillation should be considered. Epinephrine is the current first-line medication for both pediatric and adult patients in VF. If epinephrine and a subsequent repeat attempt to defibrillate are unsuccessful, lidocaine or amiodarone should be considered.
Lidocaine traditionally has been recommended for shock-resistant VF in adults and children. However, only amiodarone improved survival to hospital admission in the setting of shock-resistant VF compared with placebo. In another study of shock-resistant out-of-hospital VF, patients receiving amiodarone had a higher rate of survival to hospital admission than patients receiving lidocaine. Neither study included children. Because there is moderate experience with amiodarone use as an antiarrhythmic agent in children and because of the adult studies, it is rational to use amiodarone similarly in children with shock-resistant VF/VT. The recommended dosage is 5 mg/kg by rapid IV bolus. There are no published comparisons of antiarrhythmic medications for pediatric refractory VF. Although extrapolation of adult data and electrophysiologic mechanistic information suggest that amiodarone may be preferable for pediatric shock-resistant VF, the optimal choice is not clear.
Pediatric Automated External Defibrillators
Automated external defibrillators (AEDs) have improved adult survival from VF. AEDs are recommended for use in children 8 years or older with cardiac arrest. The available data suggest that some AEDs can accurately diagnose VF in children of all ages, but many AEDs are limited because the defibrillation pads and energy dosage are geared for adults. Adapters having smaller defibrillation pads that dampen the amount of energy delivered have been developed as attachments to adult AEDs, allowing their use in children. However, it is important that the AED diagnostic algorithm is sensitive and specific for pediatric VF and VT. The diagnostic algorithms from several AED manufacturers have been tested for such sensitivity and specificity and therefore can be reasonably used in younger children.
When Should Cardiopulmonary Resuscitation be Discontinued?
Several factors determine the likelihood of survival after cardiac arrest including the mechanism of the arrest (e.g., traumatic, asphyxial, progression from circulatory shock), location (e.g., in-hospital or out-of-hospital), response (i.e., witnessed or unwitnessed, with or without bystander CPR), underlying pathophysiology (i.e. cardiomyopathy, congenital defect, drug toxicity or metabolic derangement), and the potential reversibility of underlying diseases. These factors should all be considered before deciding to terminate resuscitative efforts. Continuation of CPR has been traditionally considered futile beyond 15 minutes of CPR or when more than 2 doses of epinephrine are needed. Presumably in part because of improvements in CPR quality and post-resuscitation care, improved outcomes from in-hospital CPR efforts beyond 15 minutes or 2 doses of epinephrine are increasingly the norm. The potential for excellent outcomes despite prolonged CPR has been highlighted by the ECPR data noted above. Conversely, the decision to discontinue CPR prematurely is final and cannot be rescinded. In the first decade of the 21st century, there is no simple answer to the important clinical question: when should CPR be discontinued?
Respiratory System
Structural and Functional Development: Age-Dependent Variables
Airways and Alveoli
The lungs appear in the fourth to eighth weeks of gestation. At this time, the lung buds divide into the mainstem bronchi; by 6 weeks all subsegmental bronchi are present; and by 16 weeks, the number of airway generations is similar to that of adults. When airway development is complete, the terminal airways remodel and multiply to form a cluster of large saccules, or alveolar precursors, that can support gas exchange. True alveoli appear before and after birth, and the respiratory saccules are thin and septate during postnatal growth.
At birth, children have approximately 24 million alveoli; by 8 years of age, the number has increased to 300 million ( Table 79.5 ). After that, further lung growth is primarily the result of increased alveolar size. There is less elastic tissue in the neonatal lung than in the lungs of adults, and the elastin extends only to the alveolar duct. By 18 years of age, elastin extends to the alveolus and is at its maximum. It then slowly decreases over the next 5 decades. Lung compliance is integrally related to the amount of elastin; hence, compliance peaks in adolescence. It is lower in the very young and the very old. Airways close in the tidal volume range until about 5 years of age.
Newborn | 6 months | 12 months | 3 years | 5 years | 12 years | Adult | |
---|---|---|---|---|---|---|---|
Respiratory rate (breaths/min) | 50 ± 10 | 30 ± 5 | 24 ± 6 | 24 ± 6 | 23 ± 5 | 18 ± 5 | 12 ± 3 |
Tidal volume (mL) | 21 | 45 | 78 | 112 | 270 | 480 | 575 |
Minute ventilation (L/min) | 1.05 | 1.35 | 1.78 | 2.46 | 5.5 | 6.2 | 6.4 |
Alveolar ventilation (mL/min) | 385 | — | 1245 | 1760 | 1800 | 3000 | 3100 |
Dead space–tidal volume ratio | 0.3 | 0.3 | 0.3 | 0.3 | 0.3 | 0.3 | 0.3 |
Oxygen consumption (mL/kg/min) | 6 ± 1.0 | 5 ± 0.9 | 5.2 ± 0.9 | — | 6.0 ± 1.1 | 3.3 ± 0.6 | 3.4 ± 0.6 |
Vital capacity (mL) | 120 | — | — | 870 | 1160 | 3100 | 4000 |
Functional residual capacity (mL) | 80 | — | — | 490 | 680 | 1970 | 3000 |
Total lung capacity (mL) | 160 | — | — | 1100 | 1500 | 4000 | 6000 |
Closing volume as percentage of vital capacity | — | — | — | — | 20 | 8 | 4 |
Number of alveoli (saccules) × 10 6 | 30 | 112 | 129 | 257 | 280 | — | 300 |
Specific compliance: CL/FRC (mL/cm H 2 O/L) | 0.04 | 0.038 | — | — | 0.06 | — | 0.05 |
Specific conductance of small airways (mL/s/cm H 2 O/g) | 0.02 | — | 3.1 | 1.7 | 0.12 | 8.2 | 13.4 |
Hematocrit | 55 ± 7 | 37 ± 3 | 35 ± 2.5 | 40 ± 3 | 40 ± 2 | 42 ± 2 | 43-48 |
pH a | 7.30 ± 7.40 | — | 7.35-7.45 | — | — | — | 7.35-7.45 |
Pa co 2 (mm Hg) | 30-35 | — | 30-40 | — | — | — | 30-40 |
Pa o 2 (mm Hg) | 60-90 | — | 80-100 | — | — | — | 80-100 |
Pulmonary Circulation
The main axial arteries of the lungs are present at 14 weeks’ gestation. By 20 weeks’ gestation, the pattern of branching is similar to that of adults, and collateral supernumerary vessels are present. During fetal life, additional arteries develop to accompany the respiratory airways and saccules. Bronchial arteries appear between the 9th and 12th weeks of gestation. The arterial wall develops a fine elastic lamina by 12 weeks’ gestation, and muscle cells are present as early as 14 weeks of gestation. By 19 weeks, the elastic tissue extends to the seventh generation of arterial branching, and muscularization extends distally. In the fetus, muscularization of the arteries ends at a more proximal level than in children and adults. The muscularized arteries have thicker walls than arteries of similar size in adults. The pulmonary arteries are actively constricted until the latter part of gestation. In the fetal lamb, pulmonary blood flow is only 3.5% of the combined ventricular output at 0.4 to 0.7 of gestation and is just 7% near term. Immediately after birth, pulmonary blood flow increases to near adult levels. Development of the pulmonary venous system mirrors that of the arterial system. The pulmonary arteries continue to develop after birth; new artery formation follows airway branching up to about 19 months of age, and supernumerary arteries continue to grow until 8 years of age. As alveolar size increases, the acinar branching pattern becomes more extensive and complex. The arterial structure also changes as preexisting arteries increase in size; the thickness of the muscular arteries decreases to adult levels during the first year of life.
Biochemical Development
By 24 weeks of gestation, the alveolar cuboidal epithelium flattens, and type I pneumocytes become the lining and supporting cells for the alveoli. The larger type II cells, which manufacture and store surfactant, also develop at this time. Surfactant initially appears at 23 to 24 weeks’ gestation in humans and increases in concentration during the last 10 weeks of gestational life. Surfactant is released into the alveoli at about 36 weeks’ gestation, thus making normal extrauterine life possible.
Respiratory Transition: Placenta to Lung
By approximately 24 weeks’ gestation, the lungs are capable of extrauterine gas exchange. However, several important circulatory and mechanical changes must occur immediately after birth for pulmonary gas exchange to be adequate. Ventilation begins to match perfusion within the first hours of life. Initially, there is right-to-left intrapulmonary shunting through atelectatic areas of the lung, as well as left-to-right shunting through the ductus arteriosus and some right-to-left shunting through the foramen ovale. The resultant PaO 2 of 50 to 70 mm Hg indicates a right-to-left shunt that is three times that of normal adults. Transition from fetal to neonatal respiration and circulation is dynamic. Postnatally, the pulmonary vascular bed remains constricted if it is exposed to acidosis, cold, or hypoxia. If pulmonary artery constriction occurs, right-to-left shunting of desaturated blood through the foramen ovale and ductus arteriosus increases and consequently reduces pulmonary blood flow. Maintenance of this active pulmonary vasoconstriction is called persistent pulmonary hypertension of the newborn or persistent fetal circulation.
Mechanics of Breathing
For ventilation of the lungs, the respiratory muscles must overcome the lung’s static-elastic and dynamic-resistive forces. Changes in these opposing forces during postnatal development affect lung volume, the pattern of respiration, and the work of breathing.
Lung Compliance Versus Age
Lung compliance changes with age because of the changing alveolar structure, amount of elastin, and amount of surfactant. At birth, compliance is low because alveolar precursors have thick walls and decreased amounts of elastin. A deficiency of surfactant (e.g., hyaline membrane disease) further decreases lung compliance. The improved lung compliance occurring over the first years of life is the result of continued development of alveoli and elastin.
Chest Wall
The chest wall of infants is very compliant because their ribs are cartilaginous. Because of the box-like configuration of an infant thorax, there is less elastic recoil than there is with the dorsoventrally flattened thoracic cage of adults. Adults have a high proportion of slow-twitch, high-oxidative, fatigue-resistant fibers in their diaphragm and intercostal muscles. Whereas adults have 65% of these fibers in the intercostal muscles and 60% in the diaphragmatic muscles, neonates have only 19% to 46% of these fibers in their intercostal muscles and 10% to 25% in the diaphragm. Consequently, infants are more vulnerable to muscle fatigue and decreased stability of the chest wall. The net effect of the compliant chest wall and the poorly compliant lungs is alveolar collapse and lower resting lung volume (FRC). Despite this tendency for lung collapse, a child maintains a large dynamic FRC via rapid RRs, laryngeal breaking, and stabilization of the chest wall with increased intercostal tone during exhalation.
Upper Airway
The upper airways of children and adults have several anatomic differences that affect their ability to maintain a patent airway. The more anterior and cephalad position of the larynx in children makes the “sniffing position” ideal for mask ventilation and endotracheal intubation. Extreme neck extension can actually obstruct the airway. The narrowest part of the adult airway is the vocal cords. Up to the age of 5 years, the narrowest portion of a child’s airway is at the cricoid cartilage, because the posterior larynx is positioned more cephalad than the anterior larynx, which causes the cricoid ring to be an ellipse rather than a circle. By 5 years of age, the posterior larynx has descended to the adult level. An ETT that passes easily through the vocal cords of a young child may cause ischemic damage to the distal airway. The cricoid narrowing and very pliant tracheal cartilage provide an adequate seal around an uncuffed ETT. Children younger than 5 years rarely require a cuffed ETT, although some practitioners use cuffed tubes regularly in these patients.
Closing Capacity
The elastic properties of the lung closely correlate with closing capacity. Closing volume is the lung volume at which the terminal airways close and gas is trapped behind the closed airways. Large closing volumes increase dead space ventilation, which leads to atelectasis and right-to-left shunting of blood. Elastic tissues help keep the airways open, so the greater the elastic stroma in the small airways, the lower the lung volume required to close small, noncartilaginous airways. Closing volume is small in late adolescence and relatively large in the elderly and the very young. Children overcome the complications of large closing volumes and secondary atelectasis by breathing rapidly, by constant activity, and by crying. Closing volume becomes a significant problem in infants who are inactive, sedated, or anesthetized.
Resistive Forces
Neonates have small airways with high resistance or low conductance (conductance = 1/resistance). The diameter of the small airways does not significantly increase until about 5 years of age; hence, young children have elevated airway resistance at baseline and are particularly vulnerable to diseases that cause further narrowing of the airways (i.e., smooth muscle constriction, airway edema/inflammation). The normal high airway resistance of neonates and young children helps maintain FRC.
Control of Breathing
The newborn’s respiratory control is unique. Hypoxia initially increases ventilation for a short time. This increase is followed by a sustained decrease in ventilation. The response is more exaggerated in preterm neonates. In full-term infants, it disappears after several weeks. Periodic breathing is also more common in infants, particularly preterm infants, and is probably due to inadequate development of the medullary respiratory centers.
Oxygen Transport: Oxygen Loading and Unloading
Fetal hemoglobin has low levels of 2,3-diphosphoglycerate and an oxygen half-saturation pressure of hemoglobin (P 50 ) of 18 mm Hg, which is much lower than the 27 mm Hg in adults. This lower P 50 allows the fetus to load more oxygen at low placental oxygen tension, but it makes unloading oxygen in tissues more difficult. Three to 6 months after birth, fetal hemoglobin has been replaced with adult hemoglobin. The increased oxygen content of fetal hemoglobin and the increased fetal hemoglobin concentration are advantageous to the fetus because it allows an oxygen content of 20 mL of oxygen/100 mL of blood to be delivered to the brain and heart. This is the same oxygen content that adults have when breathing room air. The oxygen concentration of neonates at birth is 6 to 8 mL/kg/min. It decreases to 5 to 6 mL/kg/min over the first year of life. The decreased ventilation-perfusion ratio, the decreased P 63 of fetal hemoglobin, and the progressive anemia characteristic of infants can make it difficult to deliver adequate oxygen during the first few months of life. Infants compensate by having a cardiac output of approximately 250 mL/kg/min for the first 4 to 5 months of life.
Respiratory Failure
Respiratory failure is the inability of the lungs to adequately oxygenate and remove CO 2 from pulmonary arterial blood. There are many causes of respiratory failure, including a low environmental oxygen concentration, parenchymal lung disease, and pulmonary vascular disease. A complete history of the severity and chronicity of the respiratory problem helps formulate a differential diagnosis and an approach to treatment. Specific data should include a history of prematurity, previous airway instrumentation, previous mechanical ventilation, nonpulmonary organ dysfunction, and a family history of respiratory disease. A detailed feeding history and up-to-date growth chart may provide valuable information because growth failure may increase the need for oxygen. Usually, 1% to 2% of the total oxygen consumed is used for breathing. During respiratory illnesses, as much as 50% of the total oxygen consumption may be used for breathing. Infants and children with respiratory failure often have intercostal and suprasternal retractions, signs that the work of breathing and oxygen consumption are increased. Patients grunt during expiration in an attempt to maintain FRC. Most infants and children have tachypnea, which also helps maintain FRC by decreasing the time for exhalation. Less energy is required to breath rapidly and shallowly than to take deep breaths. Infants with respiratory failure often have cyanotic lips, skin, and mucous membranes. However, it is often difficult to recognize skin color changes unless the PaO 2 is below 70 mm Hg. Symmetry of chest movement should be noted. Differences in movement may indicate pneumothorax or blockage of a bronchus. The small thoracic volume allows easy transmission of breath sounds from one side to the other. Breath sound may be normal, even when pneumothorax is present. Abdominal distention can dramatically impede breathing in infants and young children.
Monitoring of Respiratory Function
An arterial blood gas is considered the gold standard to measure oxygenation, as PaO 2 is measured directly from blood. The percent oxygen saturation of hemoglobin can be measured directly or calculated from PaO 2 , pH, PaCO 2 , and temperature. Venous and capillary blood gases do not predict PaO 2 . Use of arterial lines has decreased in pediatric ICUs over time. Pulse oximeters are ubiquitous. Pulse oximeters can provide continuous estimations of SaO 2 when the saturation is less than 97%. This is due to the shape of the oxygen dissociation curve. Pulse oximeters pass at least two wavelengths of light through the patient and the change in the absorbance of light is compared with an algorithm that produces the oxygen saturation. In the saturation range of 91 to 97%, pulse oximeters have been shown to read higher than measured arterial saturations by approximately 1%. However, in the saturation range of 76% to 90%, pulse oximeters read higher than measured arterial saturations by approximately 5% with very wide confidence intervals. Pulse oximeters may also have poorer performance when there is decreased perfusion to the extremity with the sensor. Lastly, most pulse oximeters have difficulty detecting abnormal forms of hemoglobin, such as methemoglobin or carboxyhemoglobin, and will produce erroneous results in their presence.
Umbilical artery cannulation is common in neonates, so those caring for such children can obtain arterial blood and continuously measure arterial blood pressure. These catheters are relatively simple to insert and reasonably easy to maintain. The tip of the catheter ideally should be at or just above the level of the aortic bifurcation and below the level of the renal arteries (L2). Once the child’s condition is stable, a peripheral artery catheter should be inserted and the umbilical artery catheter should be removed. All intraarterial catheters have the potential to cause distal thromboembolic disease. Care must be taken to flush arterial catheters gently to prevent cerebral or cardiac emboli. With proper insertion and maintenance, serious complications of arterial lines are rare. They have been shown to be relatively safe for short term use. Although arteries that are cannulated for a long time may develop thrombosis in a small study by Ergaz et al. all of the infants that developed thrombosis had spontaneous resolution with sequela.
PaCO 2 is used as a measure of ventilation. An arterial blood gas may be the gold standard, PaCO 2 obtained from capillary or venous blood gases can provide valuable information. CO 2 values obtained from capnography or transcutaneous CO 2 (TCOM) can provide continuous information in a manner similar to pulse oximeters. Capnographs produce a waveform displaying exhaled CO 2 that can be either time-based or volumetric. Time-based capnography is much more common. Capnographs can either be an aspirating or nonaspirating system. An aspirating system takes gas from the ventilator circuit and measure the CO 2 . A nonaspirating system has an exhalation chamber placed in line with the ventilator circuit. The system uses an infrared light source and detector which measures the exhaled CO 2 . There is a great amount of information that can come from capnography, including the end-tidal CO 2 (ETCO 2 ) value, RR, dead space calculations, cardiac output, and detection of obstruction of the airways.
For time-based capnography, the plateau of the slope will always be lower than PaCO 2 . Elevations in ETCO 2 must be investigated as they signal a change in ventilation. For individuals with healthy lungs, this ETCO 2 to PaCO 2 gradient is usually 2 to 5 mm Hg. The gradient increases with increased dead space, abnormalities in the pulmonary vasculature, decreased cardiac output, and pulmonary over distension. ETCO 2 from capnography can be used clinically to calculate the approximate alveolar dead space by the alveolar dead space fraction (AVDSf). AVDSf = (PaCO 2 − PETCO 2 )/PaCO 2 . AVDSf is a reasonable indicator of alveolar dead space and has been shown in several pediatric patients with acute hypoxemic respiratory failure to be associated with mortality. Other valuable information is available from the waveform produced by time-based capnography. As an example, a rising upslope to the exhalation phase can indicate obstructive airways disease. Time-based capnography is more accurate with slower RRs and when there is a minimal leak around the ETT.
Volumetric capnography traces the CO 2 concentration against the exhaled volume and is appearing as a feature on some ventilators. Free-standing monitors that provide the same measure are also available. Volumetric capnography provides direct information for dead space calculations. Clinically volumetric capnography is helpful in setting the optimal PEEP. In this way, PEEP can be titrated to balance improved oxygenation through alveolar recruitment and decreased dead space by not causing overdistension. Volumetric capnography can also be used to demonstrate a response to bronchodilator therapy.
In circumstances such as high frequency ventilation (HFV), the use of transcutaneous CO 2 monitoring (TCOM) provides a continuous measure of ventilation. The TCOM module heats the skin underneath a small sensor. There is increased diffusion of CO 2 across the skin as the capillary bed dilates from the heat. The diffused CO 2 is then measured. When the TCOM is first set up, it should be calibrated against a capillary or arterial blood gas. There can be drift of calibration over time, but newer modules have improved stability. A recent study by Bhalla et al. demonstrated that transcutaneous CO 2 monitoring provides an acceptable estimate of PaCO 2 , even with low cardiac output or increased subcutaneous tissue. In their study, it did not perform well in patients with cyanotic heart disease.
The effort of breathing with or without a ventilator can be obtained by the objective measurement value of the pressure-rate-product (PRP). The pressure measure by a balloon-tipped catheter placed into the distal third of the esophagus can be used as a surrogate for pleural pressure. The PRP is the change in esophageal pressure (Pes) multiplied by the RR. PRP = Pes × RR. The PRP has been used as an objective measure of effort of breathing in studies: before and after extubation, with PEEP and obstructed airways disease, evaluating increasing inspiratory load; pressure-rate product and phase angle as measures of acute inspiratory upper airway obstruction in rhesus monkeys, evaluating effectiveness of high-flow nasal cannula; and evaluating effectiveness of noninvasive ventilation (NIV) in infants. Some ventilators can measure esophageal pressure, or it can be obtained with separate devices. We have found that the accuracy of PRP measurements is sensitive to the volume of air used to fill the esophageal catheter. In addition to measuring the PRP, the esophageal pressure is very important in measuring the pressure across the lungs or the transpulmonary pressures. Many adult studies are appearing demonstrating the benefits of titrating ventilator settings to trans-pulmonary pressure for patients with acute respiratory distress syndrome (ARDS). The transpulmonary pressure may have particular benefit in patients who are obese and requiring mechanical ventilation for respiratory failure, where the decreased compliance of the chest wall may cause practitioners to limit ventilator pressures. For adult medicine, the field of esophageal pressure monitoring and the titration of mechanical ventilation to transpulmonary pressure has recently grown significantly. The need for and goals of this type of monitoring can be best summarized in a few review articles, including one from the PleUral pressure working Group (PLUG-Acute Respiratory Failure section of the European Society of Intensive Care Medicine).
The phase of breathing or the synchrony between movement of the abdomen and the chest wall can be measured with respiratory inductance plethysmography (RIP). This noninvasive measure uses elastic bands placed around the abdomen and chest. Movement of the abdomen and chest changes the inductance of a small wire in the bands. Movement of the abdomen relative to the chest can be presented graphically or measured as the phase angle. When there is obstruction to breathing, such as with upper airway obstruction, there is a lag in the movement of the abdomen and chest wall, which is identified as an increasing phase angle. The phase angle obtained by RIP is an objective measure of the degree of upper airway obstruction and can be used to evaluate the effectiveness of therapy. This is a valuable tool for research in the area of causes and treatment of upper airway obstruction, as there is great interobserver variability in the clinician’s assessment of this process. RIP can easily be measured with free-standing devices and may have an increased role in future pediatric studies.
A great deal of information on the respiratory effort of patients receiving mechanical ventilation can be obtained from respiratory spirometry. Spirometry can display flow-volume loops, pressure-volume loops, in addition to graphs of flow-time, pressure-time, and volume-time. The characteristic shape of some respiratory flow-volume loops can help with the diagnosis of various respiratory diseases. There is a classic scooped out appearance to the exhalation portion of a flow-volume curve with obstructive lung disease. The pressure-volume loops obtained on the ventilator can be used to increase PEEP to keep lung tissue recruited above an area of potential atelectasis. This can be seen graphically as a lower inflection point on the inspiratory curve. This is where the curve moves from a flat area to an area of maximal compliance where there is the greatest change in volume for a given change in pressure. There is also an upper inflection point on pressure volume loops where overdistension of the lungs can be identified if the inspiratory pressure or volume is too great. The pressure-volume curve with over-distension looks like a bird’s beak and ventilator settings should be reduced.
There are multiple noninvasive techniques that can provide additional information on the patient’s respiratory status. Radiologic evaluation of the nasopharynx, neck, and thorax can provide meaningful information regarding the cause and severity of the respiratory dysfunction. Fluoroscopy can be used to evaluate the airways and movement of the diaphragm in an uncooperative child. Electrical impedance tomography (EIT) is a noninvasive technique that does not use ionizing radiation that can provide information on regional lung ventilation. The technique uses electrodes placed on the chest wall to measure the electrical conductivity and impedance of the lung to form a tomographic image. The images are used to determine which areas of the lungs have atelectasis, normal ventilation, or overdistension. At the moment, there is much more adult data showing uses of and management strategies with EIT, as compared with pediatrics. However, as more companies produce the machines and more adult manuscripts are published, we expect there will be an increased use of EIT in pediatric mechanical ventilation monitoring. Finally, there is a rapid growth of point of care ultrasound use in pediatrics. This has multiple benefits for the patient, as it can be provided at the bedside and does not use ionizing radiation. Lung ultrasound offers the ability to identify pneumothorax, alveolar consolidation, pneumonia, atelectasis, pulmonary edema, pleural effusions, and diaphragm movements and thickness. There are an increasing number of pediatric manuscripts identifying the benefits of lung ultrasound. In the near future, ultrasound diaphragm thickness and how it changes over time may be used to guide mechanical ventilation strategies and help predict extubation success.
Respiratory Failure
The cause of respiratory failure depends to some degree on the age of the patient. Newborn respiratory failure is often the result of congenital anomalies and immaturity of the lungs and their blood vessels. Congenital anomalies can include airway malformations, dysgenesis or malfunction of the lung or nonpulmonary organs, and abnormalities of the pulmonary vessels. Lesions of immaturity include apnea of prematurity, hyaline membrane disease, and abnormalities of surfactant production and secretion. During the perinatal period, neonates are subject to infections and stress. Persistent pulmonary hypertension can complicate neonatal pulmonary and nonpulmonary problems. These and other important causes of respiratory failure in the newborn are listed in Table 79.6 . A wide variety of disorders cause respiratory failure in older children ( Box 79.3 ). Regardless of the specific cause, respiratory failure can be categorized as hypoventilation syndromes in patients with normal lungs, intrinsic alveolar and interstitial disease, and obstructive airway disease.
Location | Congenital Abnormalities | Developmental Immaturity | Specific Neonatal Stress |
---|---|---|---|
Impaired control of ventilation | Central nervous system dysgenesis Ondine’s curse | Apnea of prematurity Intracranial hemorrhage | Drug intoxication (note maternal drugs) Sepsis Central nervous system infection Seizures |
Neuromuscular disorders | Congenital myopathies | High cervical cord injuries | |
Structural impairment | Thoracic deformities Lung hypoplasia Diaphragmatic hernia Potter syndrome Abdominal malfunction Gastroschisis Omphalocele | Severe abdominal distention Pneumothorax or other leak | |
Airway obstruction | Choanal atresia | Massive meconium aspiration | |
Upper airway | Pierre Robin syndrome Laryngeal web/cleft Congenital tracheal/laryngeal stenosis Recurrent laryngeal palsy Hemangioma Lymphangioma | Vocal cord paralysis is secondary to myelodysplasia | |
Lower airway | Tracheoesophageal fistula Lobar emphysema | Meconium/blood aspiration | |
Alveolar disorders | Respiratory distress syndrome | Bronchopulmonary dysplasia |
- 1.
Impaired control of ventilation
- ▪
Head trauma
- ▪
Intracranial hemorrhage
- ▪
Increase intracranial pressure secondary to tumor, edema, hydrocephalus, Reye syndrome
- ▪
Central nervous system infections
- ▪
Drug intoxication
- ▪
Status epilepticus
- ▪
- 2.
Neuromuscular disorders
- ▪
High cervical cord injury
- ▪
Poliomyelitis
- ▪
Guillain-Barré syndrome
- ▪
Neurodegenerative disease (e.g., Werdnig-Hoffman syndrome)
- ▪
Muscular dystrophies and myopathies
- ▪
Myasthenia gravis
- ▪
Botulism
- ▪
Tetanus
- ▪
Phrenic nerve injury
- ▪
- 3.
Structural impairment
- ▪
Severe kyphoscoliosis
- ▪
Flail chest
- ▪
Large intrathoracic tumor
- ▪
Pneumothorax or pneumomediastinum
- ▪
Large pleural effusion, hemothorax, empyema
- ▪
Severe abdominal distention
- ▪
Severe obesity (pickwickian syndrome)
- ▪
- 4.
Airway obstruction
- ▪
Upper airway
- ▪
Congenital anomalies
- ▪
Tumor, intrinsic or extrinsic
- ▪
Epiglottitis
- ▪
Croup (laryngotracheobronchitis)
- ▪
Foreign body
- ▪
Postintubation edema, granulation tissue, or scarring
- ▪
Vocal cord paralysis
- ▪
Burns
- ▪
Vascular ring
- ▪
Lower airway
- ▪
Asthma
- ▪
Bronchiolitis
- ▪
Foreign body
- ▪
Lobar emphysema
- ▪
Cystic fibrosis
- ▪
- 5.
Alveolar disorders, pneumonia
- ▪
Infectious: bacteria, virus, fungus, Pneumocystis species
- ▪
Chemical: aspiration, hydrocarbon, smoke inhalation
- ▪
Pulmonary edema: cardiogenic, near-drowning, capillary leak syndrome
- ▪
- 6.
Massive atelectasis
- 7.
Oxygen toxicity
- 8.
Pulmonary confusion
- 9.
Pulmonary hemorrhage
Hypoventilation Syndromes in Children With Normal Lungs
Causes of hypoventilation include neuromuscular disease, central hypoventilation, and structural/anatomic impairment of lung expansion (i.e., upper airway obstruction, massive abdominal distention). These clinical conditions are characterized by inadequate lung expansion, secondary atelectasis, intrapulmonary right-to-left shunting, and systemic hypoxia. Atelectasis and the secondary reduction in FRC increase the work of breathing. The child’s response to the increased work of breathing and lower lung volumes is to breathe faster with a smaller tidal volume. This pattern of breathing eventually increases the amount of atelectasis and shunting. As a result, children with intrinsically normal lungs and hypoventilation syndromes exhibit tachypnea, small tidal volumes, increased work of breathing, and cyanosis. Chest radiographs reveal small lung volumes and miliary or lobar atelectasis. Positive-pressure ventilation and PEEP quickly reverse the pathologic processes.
Primary Pulmonary Alveolar or Interstitial Disorders
Intrinsic lung disease involving the alveoli or pulmonary interstitium decreases lung compliance and increases airway closure, both of which cause atelectasis and increase the work of breathing. Edema or inflammation of the alveoli or fibrosis of the interstitium decreases lung compliance. The stiffer lung requires a greater negative intrapleural pressure for air movement, thereby increasing the work of breathing and the risk for pneumothorax.
Obstructive Airway Disease
Airway obstruction can be extrinsic or intrinsic. Intrinsic small airway obstruction commonly occurs with bronchiolitis, bronchopneumonia, asthma, and bronchopulmonary dysplasia (BPD). Airway obstruction decreases conductance and increases airway resistance and the work of breathing. Partial obstruction impedes expiration more than inspiration and causes gas trapping or regional emphysema. Complete airway obstruction results in atelectasis and right-to-left shunting of blood within the lung. Patients with disease of the small airways usually have a mixture of total and partial airway obstruction and inhomogeneous collapse and overdistention of the lung. The areas of collapse cause intrapulmonary right-to-left shunting of blood, and the overdistended areas increase the amount of dead space. If the entire lung is overdistended, compliance is decreased and the work of breathing is increased. The clinical and radiographic picture varies with the different degrees of collapse and overdistention of the lung. In summary, all causes of respiratory failure share similar pathophysiology: atelectasis and decreased FRC with intrapulmonary right-to-left shunting of blood or alveolar overdistention with increased dead space and decreased CO 2 elimination, or both. The increased work of breathing associated with all forms of respiratory dysfunction can cause fatigue and a breathing pattern that further complicates the initial process. It may also lead to apnea, hypoxia, and cardiac arrest in young children if the increased work of breathing is not quickly detected and treated.
Respiratory Care
A patient’s FiO 2 can be increased by a number of means including nasal cannula or mask. The FiO 2 can be increased up to approximately 40% with nasal cannula oxygen at 5 L flow/min. However, this high rate of flow can become uncomfortable. As room air is entrained around the cannula during inspiration the FiO 2 cannot be increased further with nasal cannula. It should be noted that the size of the patient correlates with the inspiratory volume with each breath. The larger the patient, the greater inspiratory volume is relative to flow from the cannula and the greater volume of room air that is entrained. In turn, the smaller a patient the less room air is entrained and there may be a greater impact on FiO 2 .
The FiO 2 can be increased further with a properly fitted face mask. The open holes of a venture or simple face mask allow for greater entrainment of room air as compared with a non-rebreather face mask that has no holes. A FiO 2 approaching 1.0 can be obtained with a non-rebreather face mask with an oxygen reservoir and one-way valve. Patients with respiratory distress while on the pediatric ward may temporarily require a non-rebreather mask at high flows. If there is no significant improvement to immediate interventions, arrangements should be made to transfer the patient to the PICU. Non-rebreathing mask systems can be humidified for comfort, but they don’t provide any positive pressure to the airways.
High flow humidified nasal cannula (HFHNC) oxygen can provide a higher FiO 2 and is more easily tolerated as compared with standard nasal cannula. The gas in HFHNC is heated to body temperature and near completely humidified with water vapor. HFHNC can be delivered with flow rates in pediatrics up to 2 L/kg/min. HFHNC has been shown in a number of studies to decrease the effort of breathing in critically ill children. HFHNC has been used frequently to support patients with bronchiolitis. Weiler et al. demonstrated that the lowest effort of breathing for toddlers with bronchiolitis was at greater than 1.5 L/kg/min of flow. It is unclear whether the significant benefits of HFHNC come from washing out carbon dioxide from the airways, from the generation of positive pressure, or from increases in end expiratory lung volumes. The potential for complications exists with higher gas flow rates. Air leak syndrome was reported in three patients by Hegde et al. As the use of HFHNC increases, other problems may be identified. Given that the amount of FiO 2 delivered approaches 1.0, HFHNC outside of an ED or ICU setting should be used with caution. The high degree of respiratory support provided can mask a significant degree of respiratory distress. However, with appropriate monitoring and protocols, it is possible to provide HFHNC on a general ward to specific populations such as stable patients with bronchiolitis. Franklin et al. recently published a study of children younger than 12 months of age with bronchiolitis. The use of HFHNC significantly reduced the risk of escalation of care (12%) as compared with regular nasal cannula (23%).
NIV can be supplied with continuous positive airway pressure (CPAP) or bilevel positive airway pressure (BiPAP). This is typically delivered with a tight fitting nasal or face mask that allows for the development of positive airway pressure. Most modern ventilators can be set to deliver this therapy, but specific free-standing BiPAP machines are more often used. BiPAP therapy is best for short-term use and in patients who have the ability to cough and protect their airway. It is not an absolute that the patient initiates every breath as a back-up rate can be set. However, if the patient is completely reliant on the rate set on the machine, intubation should be considered. Other indications for conversion from BiPAP to endotracheal intubation include pressure related tissue breakdown on the face from constantly wearing the mask and the need to initiate enteral feedings, as patients are typically NPO on BiPAP, increasing pressure settings on the BiPAP machine.
CPAP reduces the patient’s work of breathing by providing airway pressure, reducing atelectasis, decreasing dead space, and improving the balance of ventilation to perfusion. An initial CPAP pressure is typically 4 to 6 mm Hg and is then increased as needed and as the patient tolerates the therapy. Given that the feeling of positive airway pressure is a bit foreign, starting with lower pressures and increasing gives the patient a chance to adapt. We typically start patients on CPAP therapy for several minutes even if they will ultimately receive BiPAP. For BiPAP, the expiratory pressure is also initially at 4 to 6 mm Hg and the inspiratory pressure is set at 4 to 6 mm Hg above that. The inspiratory and expiratory pressures are adjusted, as well as the rise time to inspiratory flow. All of these changes can help the patients tolerate therapy. Given the tight seal, a FiO 2 of 1.0 can be delivered. With use of the full face mask, patients are at risk for aspiration if they vomit. BiPAP therapy is being used in status asthmaticus, and it may provide a more effective means of delivering aerosolized medication. The use of BiPAP therapy for this indication may increase over time as it is being recommended in current guidelines. BiPAP can also be used as an ongoing therapy for chronic respiratory failure for such things as central hypoventilation or restrictive lung disease. These patients may be able to receive their therapy at home and are typically followed by the hospital’s pulmonary service.
The size of ETT required should be selected carefully. One formula that estimates the appropriately sized tube for children older than 2 years is (age + 16)/4. This formula provides the internal diameter of the appropriately sized ETT. The correct size should have a slight air leak when a positive pressure of 20 to 30 cm H 2 O is applied. Serious lifelong laryngeal and subglottic damage can result from using inappropriately large ETTs, particularly in children with inflammatory lesions of the upper airway such as laryngotracheobronchitis. Because of the more flexible tracheal cartilage and the relative subglottic narrowing of children, uncuffed ETTs generally provide an adequate seal in those younger than 5 years. However, if the patient has lung disease that requires high ventilation pressure, a cuffed tube is more appropriate. Small cuffed ETTs are frequently used in the ICU in younger patients, but care should be taken to ensure that there is a small leak of air at 25 to 30 cm H 2 O. Cuffed tubes will usually eliminate the air leak around an ETT, but overinflation of the cuff may occlude venous flow and injure the airway. There are presently no data on the long-term safety of using cuffed ETTs in young children. However, there has been work by Khemani et al. that showed risk factors associated with the development of postextubation subglottic upper airway obstruction included low cuff leak volume or high preextubation leak pressure. We should take care to make sure that ETT cuffs are appropriately inflated.
With endotracheal intubation, it is important to correctly position the ETT. If correctly positioned, chest movements should be symmetric, and breath sounds should be equal when auscultated in the axillae. An electronic or colorimetric CO 2 detection system helps confirm whether the ETT is in the trachea or the esophagus. If the double lines on the ETT are at the level of the vocal cords, the ETT is usually in the correct position. Another way to correctly position the ETT is to advance it into the right mainstem bronchus and listen for breath sounds in the left axilla. Breath sounds will be diminished. Withdraw the ETT slowly. When breath sounds are heard on the left, pull the tube out an additional 1 to 2 cm, depending on the size of the child. If the breath sounds are equal, fix the tube in place. The tip of the ETT should be midway between the vocal cords and the carina on the chest radiograph. In small infants, the distance between the carina and the vocal cords is very short. It is easy to inadvertently place the ETT in the right mainstem bronchus in small infants because of this short distance. Flexion of the head moves the ETT into the airway. Extension moves it toward the vocal cords. Turning the head to the side may obstruct the tip of the ETT if it comes in contact with the tracheal wall, which may cause CO 2 retention or hypoxemia, or both (unpublished data). It is common to leave a child’s trachea intubated for 2 weeks or longer before performing a tracheostomy. This is possible with proper humidification of the inspired gases, improved endotracheal suctioning and monitoring (SaO 2 ), and excellent nursing care. Everyone caring for the child must be constantly alert to the possibility that the ETT will become obstructed by secretions or that accidental extubation or mainstem bronchus intubation will occur. Tracheostomy is indicated when children require a long-term artificial airway for mechanical ventilation, for endotracheal suctioning, or to bypass an upper airway obstruction. Accidental dislodgement of the tracheostomy tube before the tract is well healed can be life threatening. Reinsertion of a tracheostomy tube during the first 72 hours after insertion can be very difficult and can create false passages that can make it impossible to ventilate the lungs or can cause pneumothorax.
Intubation and mechanical ventilation can provide significant elevations in airway pressure as compared with NIV and a FiO 2 of 1.0. There can be regional variation to the mode of mechanical ventilation chosen, but there is likely a greater use of pressure controlled ventilation rather than volume controlled ventilation in pediatric ICUs. However, as there are no studies looking at outcomes with mode of ventilation, we cannot recommend one mode over another. With pressure-controlled ventilation, pressure is set and the tidal volume may change as the pulmonary compliance changes. With volume-controlled ventilation, tidal volume is set, and the pressure needed to deliver that may change as the pulmonary compliance changes. These are likely the two main modes of mechanical ventilation in pediatric ICUs. For the majority of intubated children who have reasonable pulmonary compliance, there are little differences between the two modes. One potential advantage of pressure-controlled ventilation for patients who are sicker with poorer pulmonary compliance is that most ventilators in this mode use a decelerating inspiratory flow pattern. This means that the flow of gas is greatest early in inspiration and then decelerates to zero flow when the peak pressure is achieved. This can result in the delivery of a larger tidal volume for a lower peak pressure as compared with the same pressure that might be required in a tidal volume mode.
There are additional modes available on modern ventilators that may have benefit for patients with lung injury. The names of the modes will differ between the ventilator manufacturers. Many will have a mode where a desired tidal volume is guaranteed and the lowest pressure necessary to achieve that is used. Terms usually given to define this mode are pressure-regulated volume control and volume guarantee . These modes may reduce the pressure used, but they work best when the patient is well sedated and not competing with the ventilator.
Neurally adjusted ventilatory assist is a newer method of triggering ventilator synchrony available on the Servo-i ventilators by Maquet. This uses a small esophageal probe that can sense the electrical activity of the diaphragm and use that activity to synchronize the ventilator. The potential benefits of improved triggering such as improved comfort, lower ventilator settings, and increased minute ventilation have been shown in some studies. Some degree of expertise is necessary with the mode, as the electrical activity of the heart can also cause auto-triggering of the ventilator.
Airway pressure release ventilation (APRV) is a mode of mechanical ventilation that is less common than pressure control or volume control. There is adult data that was recently published by Zhou et al. that early use of APRV can reduce length of mechanical ventilation. As we do know, however, children are just little adults. Lalgudi et al. recently published the results of a randomized controlled trial of APRV in children with ARDS. The trial was stopped early for increased mortality in the APRV arm. There are few published pediatric studies using this mode, and its benefits and limitations continue to be explored. Like other modes of ventilation, its use appears to be regional, and some will only consider this as rescue therapy when patients fail conventional ventilation.
To describe its use, APRV is essentially CPAP with brief, intermittent release coupled with spontaneous ventilation. The high CPAP level (Phigh) maintains alveolar recruitment and aids in oxygenation over a period of time (Thigh), and the timed release to a low pressure (Plow) minimizes resistance to expiratory flow and carbon dioxide removal. In addition, the patient is able to spontaneous breath during all phases, Phigh and Plow, potentially allowing for improved pulmonary mechanics and gas exchange. APRV differs from other modes of ventilation because it relies on an intermittent decrease in airway pressure, instead of an increase in airway pressure to maintain an open lung strategy for ventilation. Therefore, the release time (Tlow) should be set long enough to allow for an adequate tidal volume (6-8 mL/kg), but short enough to avoid alveolar collapse and atelectrauma. In summary, the operator-controlled parameters in APRV are: Phigh, Thigh, Plow, Tlow, and FiO 2 . Recommendations for implementing APRV are limited in pediatrics, and thus extrapolated from adult recommendations. Plow is initially set a zero. Phigh can be set by several methods such plateau pressures or 75% of peak inspiratory pressure; however, when transitioning from conventional modes of ventilation, Phigh is often determined by m P AW pressure formula where the m P AW is set 2 to 3 cm H 2 O above conventional m P AW : (Phigh × Thigh) + (Plow × Tlow) / (Thigh + Tlow). To determine Thigh and Tlow, first determine the total cycle time according to a normal RR range for the patient’s age (i.e., a RR of 20 yields a total cycle time of 3 seconds). Thigh will be the total cycle time minus a Tlow of 0.2 to 0.6 seconds, initially starting at 0.4 seconds (i.e., total cycle time of 3 seconds yields a Thigh of 2.6 seconds and Tlow of 0.4 seconds), or Number of cycles (RR) = 60 seconds/(Thigh + Tlow). Transitioning to APRV, like transitioning to HFOV, will take time for optimal lung recruitment. After several hours, if the patient continues to have severe hypoxemia, Thigh can be increased to aid in oxygenation. Once established Plow and Tlow usually do not require further changes; however, as lung compliance improves, Phigh and Thigh can be decreased and increased, respectively, to wean a patient toward a target of a continual CPAP of 5 to 6 cm H 2 O in preparation for extubation. APRV may be advantageous to other modes of advanced mechanical ventilation because of the patient’s ability to breathe spontaneously throughout the entire ventilatory cycle improving respiratory mechanics and reducing the need for sedation and neuromuscular blockade. However, some authors are concerned that there may be a higher incidence of cyclic alveolar collapse during airway release leading to a greater degree of atelectrauma, as compared with HFOV.
High frequency oscillatory ventilation (HFOV) is being used in pediatrics as a rescue therapy for acute lung injury (ALI) or ARDS. HFOV is a subset of HFV. Use of HFOV was first described by Lunkenheimer in 1972. A 1994 publication by Arnold et al. is the only multicenter randomized trial of HFOV. This study showed lower use of oxygen supplementation in the HFOV group at 30 days. There have been other pediatric studies showing positive benefit of HFOV such as a single center prospective study by Samransamruajkit et al. and a single center retrospective study by Babbitt et al. The conclusion that could be drawn by many studies with HFOV in pediatrics is that for disease processes with significant mortality, rescue therapy with HFOV may be appropriate and may show improved outcomes. In some of the most critically ill patients with a very high mortality such as immune compromised children with ARDS, the response to HFOV has been used as diagnostic criteria to identify survivors from nonsurvivors. Early use of HFOV has been shown to decrease mortality in patients who have undergone hematopoietic cell transplant who have developed severe pediatric ARDS. There is some adult data using HFOV from large randomized trials. The OSCAR trial was a negative study and the OSCILLATE Trial was stopped early for a potential increase mortality in the HFOV group. It is unclear whether these findings can be translated to pediatric patients. The disease processes and the condition of our patients can be very different as compared with adults. Bateman et al. used a propensity score model to reanalyze results from the Randomized Evaluation of Sedation Titration for Respiratory Failure (RESTORE) Study. Using their adjusted models, they found that early use of HFOV was associated with longer mechanical ventilation. There also was no mortality benefit to the use of HFOV. Given the results of the adult studies as well as those from Bateman et al., it is unclear if the motivation would be present to perform a multicenter HFOV trial in pediatrics. There may continue to be other indications for HFOV in pediatrics, such as air leak syndrome or congenital diaphragmatic hernia.
To describe its use, HFV is a type of mechanical ventilation where the RR of the ventilator is much greater than a normal physiologic rate. Maintaining the same minute ventilation these ventilation modes produce tidal volumes that are very small. There are several different methods, but most commonly used in pediatrics is the HFOV. This type of ventilator houses a piston that is attached to semi-rigid connecting tubing which attaches to the ETT. This circuit can be pressurized to a set mean airway pressure (MAP). The piston then oscillates up to 840 times a minute, creating small positive and negative respiratory cycles. The MAP is higher as compared with conventional ventilation, and this prevents atelectasis as well as shear forces from opening and closing alveoli with each respiratory cycle. The FiO 2 is set as in a conventional ventilator. The frequency of oscillation of the piston is adjusted to remove carbon dioxide and can vary between 6 and 14 Hz (Hz = 60 cycles/s). The amplitude of the ventilator is the distance the piston moves with each excursion, and by these excursions small breaths are propelled down the tubing. Several proposed mechanisms for the method of gas transport during HFOV have been proposed but none have been proven. Tidal volumes are dependent on the patient’s compliance, ETT size, device frequency, and device amplitude. Tidal volume is inversely related to cyclic frequency: VCO 2 = frequency × VT 2 . Transitioning from conventional modes of ventilation to HFOV, the initial power setting (ΔP/amplitude) is adjusted to visible chest “wiggle” from the clavicles to the abdomen or pelvis. MAP (m P AW ) is initially set approximately 5 cm H 2 O greater than the last m P AW on conventional ventilation, just prior to the initiation of HFOV. Traditionally, tidal volumes in HFOV are considered to be just above FRC; however, it is difficult to measure actual tidal volumes and provide precise “optimal” lung volumes strategy. Clinically, MAP m P AW is titrated by upward by 1 to 2 cm H 2 O till oxygenation improves, and inspired oxygen concentrations can be weaned to less toxic levels, below 0.60. While titrating m P AW , it is important to assess for overdistention by following chest radiographs (CXR): overdistention/hyperinflation is determined by greater than nine posterior ribs or flattened hemi-diaphragms on CXR. Initial frequency settings, measured in Hz, can be found in Table 79.7 . HFOV is the only mode of ventilation with active expiration. If hypercarbia, despite allowances for permissible hypercapnia, leads to profound respiratory acidosis and patient instability, minute ventilation can be improved by several means during HFOV. First, one of the drawbacks of HFOV is the lack of spontaneous ventilation and adequate airway clearance; therefore, inline suction (without breaking into the circuit causing derecruitment) should be used to ensure adequate airway, ETT patency, and lung recruitment. Second, ΔP should be increased to maximize lung recruitment and increase minute ventilation. Third, frequency (Hz) can be slowly decreased to enhance lung recruitment and increase minute ventilation. Finally, the ETT cuff should be deflated to allow additional escape of CO 2 around the ETT. Disadvantages of HFOV include no partial ventilatory support leading to increased requirements for sedation and paralysis, cardiopulmonary interactions due higher m P AW and decreased venous return, and loss of alveolar recruitment if circuit detached for suctioning of manual ventilation.
Patient Weight (kg) | Initial Frequency Setting (Hz) |
---|---|
<2 | 15 |
2-15 | 10 |
16-20 | 8 |
21-30 | 7 |
31-50 | 6 |
>50 | 5 |
High frequency percussive ventilation (HFPV) pneumatically stacks subtidal volume breaths at a set rapid rate superimposed upon conventional cyclic rates. This allows for a progressive stepwise inflation of the lung to a set peak pressure while allowing for passive exhalation to a preset lower pressure. HFPV has been well-described in the inhalational injury population for its ability to safely oxygenate and ventilate with continuous pneumatically-powered high frequency percussions to facilitate clearance of airway debris. These properties may be particularly useful in pediatric patients with acute respiratory failure, by improving oxygenation and ventilation while maintaining lung protective strategies.
Pediatric Acute Respiratory Distress Syndrome
ARDS is a severe form of ALI that can result from a number of triggers that directly or indirectly injure the lung. The disease results in inflammation of the lungs, edema of the alveoli, and hypoxemic respiratory failure. Prior definitions of pediatric ARDS (PARDS) often were adapted from studies on adult patients and adult consensus conferences. Although PARDS represents a small portion of PICU admissions, it is very clinically important as it carries a very high mortality rate. In turn, PARDS garners a significant amount of attention from PICU researchers and clinicians. Since 2012, PICU intensivists had been using the Berlin definition of ARDS for management and study enrollment. Finally, in 2015 pediatrics had its own unique definition of ARDS. In 2015 the Pediatric Acute Lung Injury Consensus Conference (PALICC) completed their 2-year process of consensus meetings to provide a new definition of PARDS and guidelines for management. The PALICC group included 27 experts from 8 countries. They used peer reviewed data that was specific to pediatrics to form new guidelines. Where data were unavailable specifically for pediatrics, recommendations were adapted from adult or neonatal data as appropriate. In areas where there was no available data, expert opinion formed the basis of their recommendations. Multiple follow-up publications have provided important information in PARDS, including methodology of the group, the incidence and epidemiology, comorbidities, means of ventilator support, noninvasive support, monitoring, use of ECMO, and outcomes. The group identifies the need for future randomized control trials in PARDS.
There are several key aspects to defining PARDS. The PALICC definition of PARDS excludes neonates with perinatal-related lung disease, as it is likely a different entity. The lung injury should occur within 7 days of a known clinical insult. The hypoxemic respiratory failure should not be able to be explained by cardiac failure or fluid overload. Chest radiographs typically have new infiltrates consistent with parenchymal lung disease. There are now separate sections for the use of noninvasive and invasive mechanical ventilation. One significant difference in comparison to adult ARDS definitions is that the definition of PARDS for mechanically ventilated patients includes stratification by oxygenation index or oxygen saturation index. Oxygenation index (OI) = [FiO 2 × MAP × 100]/PaO 2 . The oxygen saturation index (OSI) = [FiO 2 × MAP × 100]/S p O 2 . Mild PARDS is 4 ≤ I < 8, as well as 5 ≤ OSI < 7.5. Moderate PARDS is 8 ≤ OI < 16, as well as 7.5 ≤ OSI < 12.3. Severe PARDS is OI ≥ 16, as well as OSI ≥ 12.3. There is additional information for patients with cyanotic heart disease, chronic lung disease, and left ventricular dysfunction. Future studies will need to address these more difficult aspects of defining PARDS.
The management of mechanical ventilation in PARDS currently can be summarized as the use of restrictive tidal volumes, increased use of PEEP, an ongoing relative tolerance of hypoxemia as long as it does not alter systemic oxygen delivery, and a tolerance of relative hypercarbia to reduce trauma from elevated mechanic ventilator pressures. The PALICC group among many others recognizes that there has never been a randomized control trial of tidal volume restriction in PARDS. There are also prior studies that have demonstrated a lower mortality with larger tidal volumes in pediatric patients. This seems to be a result of the increased use of pressure control ventilation in pediatric patients. An individual patient might be sick enough to be classified as having ARDS, but those with a milder form of the disease have better pulmonary compliance. As pressure is set on the ventilator, larger tidal volumes occur with better compliance. The PALICC guidelines do not make a recommendation as the mode of ventilation. However, the guidelines do recommend targeting the delivered tidal volume to be “in or below the range of physiologic tidal volumes for age/body weight (i.e., 5-8 mL/kg predicted body weight) according to lung pathology and respiratory system compliance.” It should be noted that PALICC guidelines do recommend adjusting the tidal volumes to the degree of disease severity. They recommend tidal volumes of 3 to 6 mL/kg predicted body weight for those patients with poor respiratory system compliance. They do believe the larger tidal volumes could be appropriate for patients with better pulmonary compliance.
Potentially, one of the more important recommendations from the PALICC group has been the increased application of PEEP. They recommended levels of PEEP of 10 to 15 cm H 2 O for patients with severe PARDS and identify that levels higher than 15 cmH2O may be needed for some patients. Given concerns for the potential cardiopulmonary interactions, they do recommend following hemodynamics closely. The application of increased PEEP may have significant importance in future PARDS studies. It has recently been shown by Khemani et al. that the use of PEEP settings lower than those recommended by the ARDS Network Protocol is associated with increased mortality in PARDS.
Some by extrapolation would consider the use of HFOV the most extreme form of tidal volume restriction. HFOV is able to prevent atelectasis by maintaining a constant airway pressure. Trauma from lung stretch is avoided by delivering tidal volumes that are less than anatomic dead space. Unfortunately, there is very little information in pediatric mechanical ventilation with no recent randomized control trials. There have been two recent adult studies, but they did not yield results supportive of HFOV. OSCAR was a negative study, and OSCILLATE was stopped early for a potential increase in mortality in the HFOV group. Adult practitioners are likely moving away from HFOV and use in pediatrics remains regionalized to a few centers that continue its use. The PALICC guidelines leave HFOV as a potential rescue therapy.
There are further adjunctive therapies for PARDS that will require future studies to confirm their potential benefit. This list would include the use of corticosteroids, inhaled nitric oxide, ECMO, prone positioning, use of neuromuscular blockade, and the use of exogenous surfactant. There is insufficient data at this time to state these therapies should be routinely used for PARDS. Inhaled NO should be used for patients who have documented pulmonary hypertension or right heart dysfunction. The most recent published pediatric study of inhaled NO shows the possibility for harm. The use of extracorporeal life support for PARDS has not been sufficiently studied to indicate it is beneficial. Further, given the complexity of the therapy, future trials may be very difficult, and to what extent ECMO is used for PARDS will vary based upon the individual institution.
As patients progress through their disease course and care is no longer increasing, the issue of weaning from mechanical ventilation and timing of extubation must be addressed. Various different strategies have been used to wean off of the ventilator to varying degrees of success. Likely one of the most effective strategies is the daily scheduled use of spontaneous breathing trials (SBTs) to assess whether a patient is ready for extubation. Faustino et al. in 2018 published a secondary analysis of the RESTORE clinical trial. Of patients requiring mechanical ventilation for lower respiratory tract disease, they found 43% passed their first extubation readiness. Of the group that passed an extubation readiness test, 66% were extubated within 10 hours. Many PICUs are using protocols to provide daily SBTs. In the right setting, the breathing trial can be performed safely by the respiratory therapy staff without physician input. In the near future, we will see an increase in computerized ventilation protocols driving care in the PICU. Without protocols, there is significant variability in usual care mechanical ventilation strategies, and even with protocols, there can be poor adherence to protocols. As a group, PICU intensivists would benefit from computer decision support and likely would accept some version of this. Future studies will be needed to determine if we can find the Goldilocks version of ventilation protocols. We need to provide not so little mechanical ventilation that the patient is struggling to breathe. Yet, we must provide not so much that there is atrophy of the diaphragm.
Principles of Lung Protective Strategies: Limiting Ventilator Associated Lung Injury
As lung injury progresses, in response to pulmonary or extrapulmonary injury, the lungs can be divided into three hypothetical regions: (1) areas with severe collapse and alveolar flooding “dependent areas”; (2) recruitable areas with alveolar atelectasis “intermediate areas”; and (3) normal lung “nondependent areas” ( Fig. 79.4 ). The goal of mechanical ventilation is to recruit intermediate areas, allowing improved gas exchange, spare normal areas of lung from ventilator associated lung injury (VALI), while giving dependent collapsed regions of lung with alveolar flooding time to recover from the primary process resolves (i.e., pneumonia, sepsis, etc.). Lung recruitment of intermediate areas and prevention of VALI is accomplished by using PEEP and limiting tidal volume and plateau pressures. This can be a complicated task, so let us first look at Fig. 79.5 , and try to conceptualize a theoretical pressure-volume curve of the lung. As alveolar airway pressure increases, there is an opening pressure (Pflex) required to overcome airway resistance and alveolar compliance (Compliance = ΔV/ΔP). Pressures below Pflex will lead to alveolar collapse, termed atelectasis . If airway pressure cycles above and below Pflex, alveoli will continually open and collapse, leading to wall shear stress and eventual damage: atelectrauma. Following the hysteresis curve to the upper extent of the inspiratory limb, as pressure increases, there comes a point termed, Pmax, whereby the alveoli start to become overdistended. Above Pmax, shear stress again leads to alveolar damage, this time termed volutrauma . Therefore, in theory, we attempt to keep tidal volumes on the most compliant part of the volume-pressure curve, above Pflex and below Pmax, leading to the idea called “open lung ventilation.” Triggered by the ARDSnet’s initial study, the use of low tidal volumes (6-8 mL/kg) with the addition of PEEP (open lung strategy) may reduce morbidity and mortality in patients with ARDS ( Fig. 79.6 ). However, as lung injury to the normal and intermediate areas of the lungs progresses, the volume-pressure curve moves to the right as the compliance of the lungs decreases, leaving a smaller therapeutic window requiring an increase in PEEP, resulting in higher MAP to maintain recruitment of areas of normal and recruitable lung ( Fig. 79.7 ).




Lung protective strategy attempts to decrease VALI by inhibiting volutrauma, barotrauma, atelectrauma, oxygen toxicity, and biotrauma ( Fig. 79.8 ).
Low Tidal Volume : Despite using usual control groups in the ARDSnet original study, employing a low tidal volume approach of 6 to 8 mL/kg has become a standard of care.
PEEP : The advantages of PEEP as a distending pressure include: increase in FRC, improvement of respiratory compliance, improvement of ventilation/perfusion mismatch, redistribution of lung water/edema. PEEP ultimately improves arterial oxygenation. The use of low tidal volumes has been consistent across multiple recent trials, but the selection of PEEP in these trials has been highly variable. Recent pediatric studies have shown that setting PEEP lower than the ARDSnet guidelines can result in increased mortality. Further, guidelines from the PALICC recommends increased levels of PEEP. It can be difficult to determine the critical opening pressure of alveoli clinically. Therefore, most clinicians initially use a minimal distension strategy by setting PEEP between 5 and 9 cm H 2 O, as lung injury progresses and hypoxemia worsens, PEEP may be increased to increase mean plateau pressures (keep <30-35 cm H 2 O) and aid in recruitment. More precise methods of determining optimal PEEP in patients with ARDS include titration using dynamic compliance or static pressure-volume loops to identifying the critical opening pressure. Alternatively, one can use titration with dynamic compliance or static pressure-volume curve. Likely, in the future we will determine optimal PEEP in patients with ARDS use transpulmonary pressures with an esophageal catheter. It is important to remember that as PEEP is increased and lung is recruited, intrathoracic pressure is also increased. There has been concern by clinicians that increased intrathoracic pressure can inhibit cardiac output by reducing venous return. There have been a number of studies that have shown even with increasing PEEP there is not a decrease in cardiac output. However, given the concern for decreased cardiac output, hemodynamics should be monitored as PEEP is increased.
Plateau Airway Pressures: Sustained plateau airway pressures greater than 35 cm H 2 O can lead to barotrauma: pneumothorax, pneumomediastinum, and subcutaneous emphysema. In an attempt to limit barotrauma, PaCO 2 is allowed to increase in the face of inadequate minute ventilation. This permissible hypercapnia can be employed as long as the patient tolerates the acidosis and is able to buffer with the renal retention of HCO 3 and there are no contraindications from coexisting disease.
Driving Pressure: Driving pressure is the peak inspiratory pressure minus the PEEP for patients who are paralyzed and receiving mechanical ventilation. Amato et al. demonstrated in a reanalysis of adult ARDS patients involved in prior randomized trials that decreases in driving pressure due to changes in ventilator management were strongly associated with increased survival.
Neuromuscular Blockade: Neuromuscular blocking agents (NMBA) have been provided to patients with ARDS to control ventilation and reduce trauma caused by increased tidal volume or increased airway pressure. NMBAs may also allow patients to tolerate higher levels of PEEP. This would lead to decreased trauma from repeated opening and closing of lung units. Further, NMBAs may reduce oxygen consumption from both skeletal and respiratory muscles. Papazian et al. as part of the ACURASYS study demonstrated that early use of NMBA improved the adjusted 90-day survival with ARDS and increased the time off of the ventilator. They did not see an increase in muscle weakness with the use of cisatracurium. Pediatric trials looking at use of NMBAs have not yet been performed.

Adjuvant Therapies For Acute Respiratory Distress Syndrome
Prone Positioning
In pediatric patients, Curley et al. demonstrated that prone positioning was associated with an improvement oxygenation in patients with ARDS. This group also showed proning can be safely applied in pediatric patients. However, proning was not associated with a benefit in mortality or ventilator-free days, and the trial was stopped early for futility. Prone positioning improves lung recruitment in select patients with ARDS. This may explain the difference between adult and pediatric results. In adults, Guerin et al. performed a multicenter prospective randomized control trial, Proning Severe ARDS Patients (PROSEVA), which showed that early administration of prone positioning significantly decreased 28-day and 90-day mortality. Between these two studies, the pediatric patients were more heterogenous and the adults were homogenous with more severe ARDS. The impact of patient selection and duration of prone positioning is unclear; however, it is likely that a subgroup of patients, respond to prone positioning early after lung injury, and immediate responders may benefit from prolonged proned positioning. Pediatric patients with brain injury and worsening hypoxemia prone positioning may be attempted with careful monitoring of ICP. Future studies in pediatrics are needed.
Surfactant Therapy
Exogenous surfactant therapy is standard of care in neonates suffering from RDS; however, the utility of surfactant therapy in the treatment of ALI and ARDS is uncertain and continues to be researched. In pediatric patients, a 2003 study by Möller et al. showed immediate improvement in oxygenation and a trend toward improved survival with the exogenous surfactant therapy, calfactant. However, Willson et al. in a recent randomized control trial found that surfactant did not improve oxygenation and decrease mortality relative to placebo administration. However, there was no difference in length of mechanical ventilation or ICU stay. The current PALICC guidelines do not recommend routine use of surfactant. Clinicians still feel that there still may be a specific pediatric patient population (i.e., near drowning victims) that still may benefit from exogenous surfactant administration, and research is ongoing to determine patient selection, timing, and combination with other therapies for treatment of ALI/ARDS.
Corticosteroids
A great percentage of pediatric patients with ARDS will receive corticosteroids at some point during their hospitalization. There are a number of underlying issues of inflammation and potentially adrenal insufficiency that would prompt clinicians to provide them. There were two pediatric studies published in 2015 that provide some insight. Drago et al. published a small randomized placebo controlled trial of 35 patients with ARDS. There was no difference between groups with regard to duration of mechanical ventilation, length of ICU stay or mortality. They did find that it was feasible to provide low dose infusions of methylprednisolone without significant increases in nosocomial infections or serum glucose. Yehya et al. reported a single center observational study in which 169 of 283 children with pediatric ARDS received corticosteroids for greater than 24 hours while receiving mechanical ventilation. The group that received steroids had fewer ventilator-free days and a longer duration of mechanical ventilation in survivors. Currently, the PALICC guidelines do not recommend routine therapy with corticosteroids for pediatric ARDS.
Nitric Oxide
Inhaled nitric oxide (iNO) acts as a selective pulmonary vasodilator to improve V/Q mismatch, decrease pulmonary hypertension, and reduce right ventricular cardiac work. NO upregulates cGMP, ultimately resulting in smooth muscle relaxation and pulmonary arteriolar vasodilatation. iNO is delivered directly to ventilated lung units and improves perfusion in these areas of lung without significant effects on the rest of the pulmonary vascular bed, thereby improving V/Q mismatch and oxygenation in patients with ARDS. Much like prone positioning and surfactant therapy, NO can result in transient improvements in oxygenation. A systemic review and meta-analysis performed by Adhikari et al. in adults with ARDS showed inhaled NO does not reduce mortality, regardless of severity of illness. In pediatrics, a small, randomized trial by Bronicki et al. showed that pediatric patients with ARDS randomized to receive iNO had significantly improved ECMO-free survival and ventilator-free days at 28 days. This trial only had 55 total subjects and did not show a difference in overall mortality. The most recent study was a propensity matched cohort of 499 pediatric patients with ARDS by Bhalla et al. There were a total of 143 that received iNO. They found that use of iNO was not associated with improvement in ventilator-free days or mortality. There still remains a role for iNO in patients with pulmonary hypertension, right heart dysfunction, and potentially a bridge to ECMO. Any further roles would require future studies to show benefit.
Extracorporeal Membrane Oxygenation
ECMO remains a rescue strategy for pediatric patients with ARDS that fail to respond to advanced modes of mechanical ventilation. There has been much more research with ECMO in adult ARDS as compared with pediatrics. The CESAR Trial in adults demonstrated improved outcomes in adults treated with ECMO; however, this trial had significant methodological flaws. Results of the EOLIA (ECMO to rescue lung injury in severe ARDS) were reported in 2018. The trial enrolled adults with very severe ARDS. They found that 60 day mortality was not significantly lower in the group randomized to ECMO as compared with conventional mechanical ventilation and possible use of ECMO as a rescue therapy. With regard to pediatric data, in 2018 Barbaro et al. published a secondary propensity score matching analysis of ECMO use in patients from the RESTORE study. They found that in patients with severe pediatric ARDS there was not superior outcomes in the group treated with ECMO as compared with those that did not receive ECMO support. With improved care and research, presumably the mortality risk for both ECMO as well as ARDS will decrease. J. C. Lin recently published a review on this topic which may provide additional insights. Further research regarding ECMO support of severe pediatric ARDS is needed.
Adjunctive Pharmacologic Therapy: Sedatives and Analgesics
Sedation is often required to help children cooperate with mechanical ventilation. The amount of sedation required depends on the child’s age, size, underlying disease, and amount of respiratory support needed. Some children are neurologically depressed and require no sedation. Sedation allows patients to breathe “in phase” with the ventilator, which reduces the peak airway pressure and eliminates coughing and straining, all of which can cause pulmonary gas leaks or inadequate ventilation. Sedation has changed over the past many years in PICUs across the country. Part of the cause of this can be attributed to the development of protocols to limit sedation to decrease tolerance, withdrawal symptoms when sedation was weaned, and the potential for exacerbation of delirium. Many of the larger PICUs participated in the RESTORE study. The RESTORE study can serve as a good example of how to deliver sedation using protocols. Each patient’s level of sedation was titrated to a State Behavioral Scale, which allowed for a common language among care providers. The medications that they used to provide sedation while patients were intubated were primarily morphine and midazolam. They recommended the use of fentanyl infusions as the opioid agent for patients that had hypotension or reactive airways disease. There were also secondary sedation agents that were used which included pentobarbital, ketamine, methadone, clonidine, dexmedetomidine, and propofol. Some institutions did use lorazepam as an alternative benzodiazepine. Pentobarbital and ketamine were adjunct medications when the patient was unresponsive or less responsive to the primary agents. Methadone and clonidine were adjuncts used primarily to prevent withdrawal symptoms as the patient was tapered off medications. Dexmedetomidine and propofol were used in this study as temporary agents to wean off other medications in anticipation of extubation. Propofol is not used in some units or only used as a temporary measure because of the concerns for propofol infusion syndrome. Dexmedetomidine is seeing increased usage in PICUs across the country not just for short-term sedation. In some instances, it can replace opioids as a means of providing sedation. There should be a word of caution in administering lorazepam to premature neonates. Lorazepam repeatedly dosed over several days may cause steroid-responsive hypotension as a result of buildup of drug in the body. The half-life of lorazepam is approximately 72 hours in premature infants. Giving the drug every 4 to 6 hours causes accumulation of the drug in blood and tissues.
NMBAs increase chest wall compliance, reduce oxygen consumption, and facilitate mechanical ventilation. If neuromuscular blocking drugs are used, they should be administered in conjunction with medications that cause amnesia and anxiolysis and control pain. Vecuronium, rocuronium, and cisatracurium are the most commonly administered muscle relaxants used in the PICU (also see Chapter 27). Rocuronium is often given for intubation and for intermittent dosing. Vecuronium can be used as an intermittent agent but also as a continuous infusion. There are risks of decreased metabolism and prolonged clearance with vecuronium infusions and many will choose instead to use cisatracurium. Cisatracurium is often useful because its elimination is not dependent on renal or hepatic function. If these drugs are given for more than a day, provision of regular drug holidays should be considered to avoid serum buildup of the drug and prolonged paralysis.
Weaning from Mechanical Ventilation
The pediatric literature falls behind adult publications with regard to guidance in weaning and extubation from mechanical ventilation. A 2009 review by Newth et al. addressed what was known at the time. Attempts have been made to develop predictive indices identifying which children can be successfully weaned from mechanical ventilation. Most of the indices are used in research but do have clinical applications. One indices is the Rapid Shallow Breathing Index (RSBI) from Yang and Tobin. The RSBI is equal to the RR/tidal volume. When patients are breathing comfortably, they have a lower RR with larger tidal volumes. In this circumstance, the RSBI is a low number. Further, patients with respiratory distress tend to breathe more rapidly with smaller tidal volumes and hence have a higher RSBI. There are several different techniques for weaning from mechanical ventilation. These include lowering the set ventilator rate over time, performing a daily SBT, or increasing pressure support or CPAP sprints. Many hospitals are initiating daily SBTs for their patients. These are periods of decreased ventilatory support while the patient is observed for evidence of respiratory distress. The decreased support can be pressure support, CPAP, or T-piece ventilation. Patients are observed to see that there is no significant increase in RR, decrease in saturations, diaphoresis, hemodynamic compromise, or evidence of increased work of breathing. The successful completion of a SBT will initiate of plans for extubation. Depending on the ICU, SBT are carried out under direction of the physicians or they can be independently performed by the respiratory therapist. It is possible that computerized weaning protocols will further reduce time on mechanical ventilation. At the moment it seems that the best solution is to ask on a daily basis whether the patient is able to be extubated. Studies indicate a significant percentage of patients being evaluated for weaning are ready for extubation.
We must keep in mind that as we are weaning patients from mechanical ventilation, there is an anticipated extubation failure rate. If we continue mechanical ventilation until we are absolutely certain a patient will not fail, many patients will be ventilated longer than necessary. A 2003 review of 16 ICUs showed an extubation failure rate of 6.2% (range 1.5%-8.8%) for patients requiring mechanical ventilation longer than 48 hours. Our own research shows a reintubation rate of 8.3% following extubation.
With regard to the degree of decreased ventilatory support during an SBT, use of CPAP does not put patients under increased stress. Multiple prior studies demonstrate that infants or children with smaller ETTs are not “breathing through a straw” when put on CPAP or T-piece. Relative to an adult, the ETT may be smaller in diameter, but it is also shorter and the inspiratory flow rate in infants is low compared with an adult. Flow rates are measured to be approximately 0.5 L/kg/min. Therefore, a 3 kg infant has a flow velocity of 1.5 L/min as compared with 30 L/min for a 60 kg adult. If patients are unable to successfully complete an SBT, it is possible they would not manage the work of breathing postextubation.
Overall, the criteria for extubation readiness includes intact airway reflexes, hemodynamic stability, manageable secretions, and an appropriate level of alertness. The amount of negative inspiratory force (NIF) the patient can generate can be measured. A NIF should be measured with a calibrated manometer and inspiration from residual volume. Typically, a NIF of negative 30 or more is associated with extubation success. The presence of a leak around the ETT may be supportive of extubation. There is some research showing the absence of a leak around the ETT does not predict extubation failure. However, absence of a leak is associated with the development of subglottic upper airway obstruction after extubation. Extubation failure is typically defined as reintubation less than 24 hours after a scheduled extubation attempt. Extubation failure may be caused by a multitude of reasons but large category is postextubation upper airway obstruction. We believe the incidence of upper airway obstruction to be between 37% and 41%. As there is significant interobserver variability in the clinical assessment of upper airway obstruction, physiology based tools may be valuable. Objective measures of airway obstruction may be necessary before we have a definitive answer as to which therapies reduce subglottic narrowing and reduce obstruction.
Respiratory Disorders
Laryngotracheobronchitis (Croup)
Croup occurs most frequently in the 3-month-old to 3-year-old age group. Croup is a viral infection (parainfluenza, influenza, adenovirus) that causes edema of the tissues in the upper airway, particularly in the immediate subglottic region. There is usually a history of a few days of upper respiratory tract infection symptoms followed by hoarseness, a croupy cough, and possibly stridor. The degree of respiratory distress and the child’s ability to compensate for the increased work of breathing are best assessed clinically. These patients are initially treated with mist and aerosolized racemic epinephrine to reduce swelling of upper airway mucosa. Steroid administration is common yet controversial. Tracheal intubation is required when the child can no longer sustain the increased work of breathing and CO 2 increases. When the trachea is intubated, the ETT should be 0.5 to 1.0 mm smaller than usual for age. The ETT must be of sufficient size that the patient can breathe easily during spontaneous ventilation and the nurses can effectively suction secretions from the airways. Croup usually resolves spontaneously in 3 to 7 days. The average duration of tracheal intubation is approximately 5 days. Laryngotracheobronchitis is less common in children older than 4 years.
Epiglottitis
Epiglottitis is an inflammation of the mucosa of the supraglottic structures that was previously caused by Haemophilus influenzae type B but is now commonly caused by Staphylococcus aureus and streptococcal organisms because immunization against the Haemophilus organism has been so effective. In the past, epiglottitis was a disease of 4- to 6-year-old children. Now, it occurs more commonly at older ages (even in adults). In young children, epiglottitis is a true airway emergency because it may rapidly progress to complete and fatal airway obstruction. Providing a secure airway is the first priority. Children with epiglottitis appear toxic and have a fulminant onset of fever and respiratory distress. Tracheal intubation during anesthesia is commonly required until antibiotic therapy (ampicillin and chloramphenicol or ceftriaxone) is initiated and the signs of systemic toxicity subside. Introduction of the H. influenzae vaccine has dramatically decreased the incidence of H. influenzae infection, as well as that of other H. influenzae processes.
Bronchiolitis
Bronchiolitis is an acute viral infection of the lower respiratory tract that occurs primarily during the first 2 years of life. The signs and symptoms of this malady are air trapping, wheezing, mild to moderate systemic hypoxemia, increased work of breathing, and increased airway resistance. The cause is usually an infection with respiratory syncytial virus (RSV). Patients at high risk for respiratory failure from this disease include infants and young children with a history of prematurity, chronic lung disease, or congenital heart disease. Apnea is frequently the first sign of decompensation in neonates, which often occurs before significant hypercapnia appears. Fatigue is a common indication for mechanical ventilation. Treatment is primarily supportive and includes endotracheal intubation and mechanical ventilation for respiratory failure. RSV immune globulin (RespiGam) is a prophylactic IV drug that is administered to patients at risk for seasonal RSV disease (ex-premature infants, infants with congenital heart disease, immunosuppressed patients, or those with multiple congenital anomalies); it has dramatically decreased the incidence of illness in this population. Ribavirin is a virostatic drug available for the treatment of children with RSV and congenital heart disease, immunosuppressive disease, or multiple congenital anomalies.
Cystic Fibrosis
Cystic fibrosis is a fatal autosomal recessive disease carried on chromosome 7. Although it affects the pancreatic, hepatic, pulmonary, GI, and reproductive systems, 90% of the reported morbidity and mortality is pulmonary. The pulmonary pathology consists of severe airway obstruction, bronchiectasis, emphysema, and terminal respiratory failure.
Survival of patients with cystic fibrosis has improved dramatically over the past 30 years. More than one-third of patients survive to greater than 30 years of age. This change is the result of improved antibiotic therapy, nutritional support, and aggressive treatment of complications. Lung transplantation has been performed in patients with chronic respiratory failure with varied success.
Bronchopulmonary Dysplasia
BPD is a form of chronic lung disease that occurs in patients who have survived severe neonatal lung disease. The cause of this disease is uncertain, but usually patients were premature, had hyaline membrane disease, and required prolonged and aggressive respiratory support with high inflating and distending pressure and high oxygen concentrations. Inflammation appears to be an important component of this disease. Children with BPD have decreased dynamic compliance, increased airway resistance, increased physiologic dead space, and markedly increased work of breathing. The lungs are hyperinflated, and there are intercostal retractions, nasal flaring, and wheezing. Chest radiographs demonstrate large lung volumes and fibrosis as well as cystic and atelectatic areas. Hypercapnia and hypoxia are present to varying degrees. Therapy for BPD includes providing enough calories to meet the higher energy expenditure required for the increased work of breathing. Respiratory support (mechanical ventilation, CPAP) is required for some patients. Diuretics and bronchodilators are frequently used and may be associated with electrolyte abnormalities. Most long-term survivors have subjectively normal pulmonary function. However, some survivors have severe chronic physiologic changes. During the first years of life, viral or bacterial pulmonary infections often increase the need for respiratory support; these infections may prove to be fatal. Great effort is being made to prevent BPD. Because the trauma of mechanical ventilation on an immature lung is thought to be the major cause of BPD, alternative modes of ventilatory support are being evaluated, including exogenous surfactant, high-frequency ventilation (especially HFOV), ECMO, and liquid ventilation.
Sleep Apnea
Normal ventilation during sleep requires normal upper airway anatomy and normal intact reflexes. The latter include central responses to hypercapnia and hypoxia, response to airway irritation, and dynamic phasic contraction of the pharyngeal and hypopharyngeal muscles. Sleep apnea usually occurs when one or more of these protective responses is abnormal. In infancy, sleep apnea is relatively common. Numerous causes have been postulated, the most attractive of which is immaturity of the medullary chemoreceptors. Patients with Ondine’s curse, the most severe form of central apnea, have complete apnea when asleep. Lesser breathing disorders may be present during sleep in patients with sudden infant death syndrome. Treatment of sleep apnea consists of respiratory stimulants (theophylline), cardiorespiratory monitoring during sleep, and in some severe cases, tracheotomy and nighttime mechanical ventilation. Obstructive sleep apnea occurs in all pediatric age groups. It may be associated with an identifiable anatomic disorder, such as enlarged tonsils and adenoids, the Pierre Robin syndrome, and tracheal and laryngeal malacia. Signs and symptoms of obstructive sleep apnea include loud snoring, obstructive episodes with frequent arousal, behavior disorders caused by sleep deprivation, and cor pulmonale. The diagnosis is made by the history, ECG, and formal sleep studies. Bronchoscopy may also be helpful in defining the problem. In young children, an enlarged liver may suggest that the child has pulmonary hypertension. Treatment of sleep apnea includes removal or bypass of an obstruction. Although tonsillectomy and adenoidectomy may improve the airway, there may still be significant airway obstruction for the first few days after surgery. Rarely does such a child require a tracheostomy.
Foreign Body Aspiration
Foreign body aspiration is a relatively common and often catastrophic event in children. Although it can occur at any age, the peak incidence of foreign body aspiration occurs at 6 months to 3 years of age. Vegetable matter (peanuts) and other foodstuff (hot dogs) or coins and small pieces of toys are the most commonly aspirated material. Many of these objects are radiolucent. The symptoms of aspiration are related to the location of the foreign body within the airway and the time since the object was aspirated. Acute symptoms may include total airway obstruction, stridor, wheezing, or acute onset of coughing, whereas more chronic symptoms include bloody sputum, chronic coughing, or wheezing. The diagnosis of a foreign body in the airway is made from the history and physical examination and, in some cases, by radiologic imaging. The efficacy and safety of abdominal thrusts have been questioned. The Heimlich maneuver and back slaps are reserved for acute upper airway obstruction with no air movement. Treatment of less acute or lower airway foreign body aspiration includes bronchoscopy, postural drainage, chest physical therapy, bronchodilators, and surgical removal of the object.
Upper Airway Obstruction and Meningomyelocele
Vocal cord paralysis is a common result of several disorders, including brainstem abnormalities and myelodysplasia. Children with meningomyelocele usually have an Arnold-Chiari malformation and may have stridor. This lesion allows caudal displacement of the medulla, stretching of the cranial nerve tracts, and abnormal arterial architecture of the brainstem. Vocal cord paralysis may result from pressure on the brainstem (i.e., with hydrocephalus) or from focal infarcts of the brainstem. Treatment of these brainstem abnormalities includes relief of the hydrocephalus and, if the paralysis persists, cervical decompression of the Arnold-Chiari lesion. Despite these surgical procedures, some children require a tracheotomy and long-term mechanical ventilation.
Asthma
The prevalence of childhood asthma is increasing over time. Estimates from the Centers for Disease Control and Prevention placed the percentage of children with asthma at 3.6% in 1980 5.8% in 2003 and 9.5% in 2011 ( www.cdc.gov/nchs/fastats/asthma.htm ). Thankfully, the majority of children with asthma will never require intensive care. However, for the children that do there can be significant morbidity and a risk of mortality. In a 2012 study by Newth et al. of fatal and near-fatal asthma in an ICU setting, 12% of patients had complications and the mortality was 4%. Cardiac arrest prior to admission occurred in 10 of the 11 patients who died. For the purposes of this section critical asthma is defined as an asthma exacerbation requiring ICU admission.
Asthma is a disease of inflammation. There is infiltration of the submucosal area of the airways with mast cells, eosinophils and CD4 lymphocytes. Degranulation of mast cells releases leukotrienes and histamine causing edema, increases in mucus production, and chemotaxis of white blood cells (WBCs). Multiple factors can trigger an asthma attacks and degranulations of mast cells. Such factors include allergens, infections (viral > bacterial), changes in the weather, and strong emotions. Inflammation increases irritability of the airway as well as airway hyper responsiveness. The airway lumen is decreased by spasm of the bronchial muscles, edema, and mucus. Decreases in the airway lumen significantly increase airway resistance. For laminar airflow, airway resistance is related to the third power of the radius, but with turbulent airflow it is related to the fourth power. Due to the smaller lumen of their airways, children experience much greater changes in airway resistance during asthma attacks than adults. Due to resistance in exhalation, an expiratory wheeze is typically noted. Bronchospasm, edema, or mucus plugging can lead to complete obstruction of small airways. Hypoxemia occurs as a result of a mismatch in ventilation and perfusion. Airway dead space is increased by obstruction of the airways. To maintain ventilation, the RR is typically increased. In turn, the initial PaCO 2 is usually low. Respiratory fatigue or impending failure may be identified by a normal or elevated PaCO 2 .
It should be reinforced early that wheezing is caused more things than just asthma and that asthma can occur without wheezing. Wheezing is the sound that is produced when there is obstruction to airflow. Wheezing can be caused by pneumonia, upper airway obstruction, foreign body aspiration, and CHF. Each would require different therapy. Wheezing in a toddler of a sudden onset should prompt evaluation for foreign body aspiration. The history should be addressed at recent choking or coughing episodes. Even the presence of a history of reactive airways or atopy does not rule out aspiration. A high index of suspicion should be maintained. Intermittently a chest-ray obtained for a child who is wheezing shows cardiomegaly rather than peribronchial cuffing. Asthma is statistically more likely but this can be the presentation of heart failure. In turn, a chest x-ray is warranted for any patient with their first presentation of wheezing patient and certainly for any child who is admitted to the ICU for wheezing. With regard to asthma that occurs without wheezing, the movement of air is required to hear wheezing. It is possible to have such significant airflow restrictions that patients don’t produce audible wheezing. Patients who present with a quiet chest or limited airflow on auscultation require immediate action.
Children presenting with an asthma exacerbation may have a few days of URI symptoms followed by increased work of breathing. The patient may have low room air oxygen saturations. The patient’s position of comfort may be sitting up to support their muscles of respiration. Accessory muscle use is noted. There is a prolonged expiratory phase on auscultation. Some children in an effort to increase airway pressure may be breathing out with pursed lips or in smaller children grunting may be noted. The child may not be able to speak in more than one or two word phrases. Therapy should be started immediately first with supplemental oxygen to address hypoxemia. If the child mild respiratory distress, nasal cannula oxygen may be sufficient. If the child has moderate or severe respiratory distress, oxygen should be delivered by either face mask or non-rebreather face mask. Inhaled beta agonists such as albuterol are given to relax bronchial smooth muscles. IV or subcutaneous terbutaline or epinephrine may be required if there is not enough air movement to deliver inhaled medications. Steroids should be given early as the effect will take some time. If there is limited improvement with initial therapy arrangements should be made for ICU admission. Many emergency departments will attempt to obtain arterial blood gases. However, the clinical picture alone may provide enough information to guide therapy.
Asthma Therapy
Supplemental Oxygen
Oxygen can be delivered with a standard nasal cannula, but the FiO 2 is limited. Standard nasal cannula might provide up to 28% FiO 2 . Increasing the flow of oxygen with a standard cannula above 4 to 5 LPM is not usually tolerated. A simple face mask might increase the FiO 2 to 50%. A tightly sealed non-rebreather face masks might be able to provide a FiO 2 closer to 1. Providing oxygen with a HFHNC may provide nearly complete humidification of the airway gases and the FiO 2 also approaches 1. A 2014 study by Rubin et al. demonstrated a decrease in effort of breathing in patients receiving HFHNC. The mechanism of its effect is not elucidated. Some clinicians have begun using HFHNC as a mechanism for delivery of beta agonists or other aerosolized medications, but there is not yet data supporting its use.
Inhaled β Agonists
Inhaled β agonists are used to relax bronchial smooth muscles. The most commonly used β agonists is albuterol, which is a racemic mixture of the active R and inactive S enantiomer. Levalbuterol, the active R enantiomer, is available as a separate preparation, but recent studies have not shown it to be more effective or have less elevations in HR. Albuterol is β 2 selective and comes as a metered dose inhaler or solution for nebulization. For initial therapy in the ICU, continuous albuterol is preferred, and the usual dose is 0.15 to 0.5 mg/kg/h or 10 to 20 mg/h. After improvement in air movement with decreased respiratory distress, intermittent doses can be given every 1 to 2 hours. Terbutaline as an inhaled medication is less β 2 selective compared with albuterol and therefore is used less commonly. Terbutaline remains as an important IV medication. Tachycardia is commonly associated with albuterol use. At times it is unclear if the elevated HR is related to toxicity of medications or due to ongoing respiratory distress. Arrhythmias may be seen with albuterol use but this is usually just an increased frequency of PVC. Diastolic hypotension is observed with higher doses of albuterol, although this can be related to decreased intravascular volume and increased intrathoracic pressure. Agitation and tremors can be seen with CNS stimulation. Hypokalemia may be noted as the Beta agonists drive potassium into cells. Ipratropium bromide, an inhaled anticholinergic, is sometimes paired with intermittent albuterol doses. Ipratropium bromide has the benefit of promoting bronchodilation without decreasing mucociliary clearance.
Corticosteroids
IV steroids are preferred to oral dosing in the ICU due to the possibility of decreased absorption or delayed onset. Methylprednisolone is used commonly due to its limited mineralocorticoid effects. The initial dose is 2 mg/kg and then 0.5 to 1 mg/kg every 6 hours. There may be regional preference for dexamethasone or hydrocortisone. Steroids are typically given for the duration of the acute asthma phase. If steroids are given for 5 days or less, they are not usually tapered. Systemic steroid use is associated with hyperglycemia, hypertension and occasionally agitation. In the initial ICU care inhaled steroids are of no benefit.
Intravenous Fluids
Children admitted to the ICU with critical asthma will likely be dehydrated from limited oral intake during their illness and increased insensible losses from the higher RR. The patient’s circulating volume should be supported with fluid boluses if needed. However, excessive fluids should be avoided as this could lead to pulmonary edema. Pulmonary edema could worsen both oxygenation and airway resistance. Fluid boluses may also be needed for children whose respiratory distress progresses require mechanical ventilation. Hypotension often occurs during intubation.
Intravenous and Subcutaneous β Agonists
A significant decrease in air exchange may result in poor delivery of inhaled medications and IV β agonists may be required. Terbutaline is often the first choice of the available beta agonists. Terbutaline is relatively β 2 selective as compared with isoproterenol and epinephrine. Terbutaline can be given subcutaneously for children without IV access as a dose of 0.01 mg/kg/dose up to a maximum of 0.3 mg. IV terbutaline is loaded at a dose of 10 μg/kg over 10 to 20 minutes followed by a continuous infusion of terbutaline in a range of 0.1 to 10 μg/kg/min. The infusion can be titrated to effect. Subcutaneous epinephrine may be given for severe asthma exacerbation with limited IV access with a dose of 0.01 mg/kg of to the 1:1000 solution with a maximum dose of 0.5 mg. Absorption of the drug may be limited by poor perfusion to extremities. IV epinephrine may be an ideal drug for mechanically ventilated patients with hypotension. The use of isoproterenol in critical asthma has decreased over time.
Methylxanthines
There is regional variation in the use of the methylxanthine aminophylline in place of IV terbutaline as a second line drug for critical asthma. Fewer children with asthma are admitted to the ICU with a methylxanthine as a chronic medication. There are improved controller medications including the leukotriene inhibitors so fewer children are receiving oral theophylline. The methylxanthines promote bronchial smooth muscle relaxation, but the exact mechanism of action is not known. IV aminophylline is loaded at a dose of 5 to 7 mg/kg over 30 minutes, followed by a continuous infusion in the range of 0.5 to 0.9 mg/kg/h. If the patient has taken oral theophylline in the past 24 hours, the loading dose is reduced by 50% or the aminophylline dosing is adjusted based on a serum theophylline level. In general, an aminophylline loading dose of 1 mg/kg will raise the serum theophylline level by 2 μg/mL. The target range for serum theophylline in acute asthma is 10 to 20 μg/mL. Theophylline has a very narrow therapeutic window. Theophylline levels above 20 μg/mL are associated with nausea, tachycardia, restlessness, or irritability. Higher theophylline levels have been associated with seizure activity.
Magnesium
Magnesium can be given as an inhaled or IV medication to relax bronchial muscles. Smooth muscle relaxation occurs due to magnesium’s effect as a calcium channel blocker. Results from the 2013 MAGNEsium Trial in Children (MAGNETIC) indicate nebulized magnesium may be beneficial in an acute severe asthma exacerbation. There is some evidence that IV magnesium may also be beneficial in severe asthma. As there is still regional variation to magnesium, at the least a magnesium level should be checked with the initial set of electrolytes and hypomagnesium should be treated. The dose for critical asthma and hypomagnesium can be the same 25 to 45 mg/kg given over 30 minutes. Magnesium toxicity can include muscle weakness, cardiac arrhythmias, decreased reflexes, and respiratory depression.
Helium
Mixtures of helium and oxygen (heliox) can be used to improve laminar gas flow. This occurs due to the decreased density of helium as compared with nitrogen (approximately one-seventh). For Helium to be beneficial in small airways, it must occur in a high ration with oxygen. The greatest benefits may be seen with an 80:20 or 70:30 ratio of helium:oxygen. Therefore, hypoxemia and the need for increased supplemental oxygen may limit heliox use. There is data supporting the use of heliox as a method of delivering inhaled β 2 agonists. As with other advanced therapy, helium may not have a role in routine care, but there may be benefit from its use in severe critical asthma.
Ketamine
Ketamine is a noncompetitive N-methyl-D-aspartate (NMDA) receptor antagonist that can produce a state of dissociative anesthesia. An additional effect of ketamine is bronchodilation. It is a useful medication for sedation in the ICU as ketamine has limited effect on the respiratory drive, and in usual doses, hemodynamics are not usually affected. For patients with asthma who are intubated and mechanically ventilated, ketamine may be a good choice for sedation along with a benzodiazepine. Further, there was one pediatric study showing an improvement in the PaO 2 /FiO 2 ratio as well as dynamic compliance in mechanically ventilated children with refractory bronchospasm who were receiving a continuous infusion of Ketamine. There has been no study to show whether ketamine used to treat anxiety is beneficial in preventing intubation in patients during an asthma attack. For nonintubated patients, a recent Cochrane Database Review did not show significant benefit in severe acute asthma. If a decision is made to use ketamine either for a procedure or a means of sedation, the bolus dose is usually 1 mg/kg, allowing time for effect before repeating. Ketamine is given as a continuous infusion at the dose of 5 to 30 μg/kg/min titrated to effect. One of the additional side effects of ketamine can be dysphoria, and as such it is usually given with a benzodiazepine.
Noninvasive Ventilation
There is very limited evidence that NIV is effective in children with asthma. Clinically patients with effective air exchange who fight the mask and positive pressure do not benefit from NIV. Nevertheless, some children with very limited air exchange and fatigue take to NIV easily and appear more comfortable. NIV may allow time for therapies to become effective (steroids) and may prevent intubation. This should not be used when the level of alertness or ability to protect the airway is diminished.
Intubation
By the time patients with asthma require intubation and mechanical, they are hypoxemic, acidotic, fatigued, and have limited reserve. It is suggested that the most experienced person available perform the intubation. Appropriate venous access is required, and fluid boluses may be necessary. Ketamine and a benzodiazepine have been recommended by many. Ketamine may produce increased secretions, so atropine should be considered. Ketamine may produce dysphoria so the benzodiazepine is given for its amnestic effects. The management style in our ICU is to get the patient back to breathing spontaneously or initiating their own breathes as soon as possible after intubation. In turn, Rocuronium can be considered as medium short-acting muscle relaxant. Succinylcholine can be used, but the other side effects of this medication, including elevation of potassium, should be considered. A cuffed ETT is recommended, as ventilator settings may require high peak pressures. Immediately after intubation, we typically hand ventilate the patient with a low rate. This is to prevent alveolar overdistension and reduce the risk of pneumothorax. There can be acute decompensation following intubation. Hypovolemia and increased intrathoracic pressures may be an issue. Following the DOPE acronym, one should also look for Displacement or Obstruction of the ETT and rule out Pneumothorax or Equipment failure.
Mechanical Ventilation
There can be controversy regarding the best means to mechanically ventilate patients with asthma. The argument against setting the ventilator in a pressure control mode is that changes in airway resistance that occur with asthma can result in delivery of an inadequate tidal volume. The argument against a the ventilator in a volume control mode is that due to flow patterns of the ventilator the same tidal volume can be delivered with a higher peak pressure as compared with a pressure control mode. As stated before, our management style is to get intubated asthmatics breathing spontaneously as soon as possible. This allows them to set their own breathing rate, and on pressure support and PEEP, they can set their own inspiratory to expiratory ratio. Pressure support has been recommended because it is patient-initiated, even if not patient-limited. There may be initial elevations in PaCO 2 , but if the patient is well oxygenated, the carbon dioxide elevation is usually well tolerated.
Historically, clinicians set zero or low PEEP for intubated asthmatics due to the perceived risk of hyperinflation and barotrauma. However, since 1988 there have been four adult studies and one pediatric study strongly suggesting the benefits of extrinsic PEEP during mechanical ventilation for intubated asthmatics. These studies demonstrated that extrinsic PEEP, up to a level to matching intrinsic PEEP, improves the triggering sensitivity of the ventilator, diminishes ventilatory work, and reduces mechanical work of breathing for patients spontaneously breathing with assisted ventilation. As the work of breathing is reduced, there is improved patient comfort and potentially a reduced need for sedation. It is felt that matching extrinsic PEEP applied with the ventilator to the intrinsic PEEP developed by the patient with asthma may improve delivery of aerosol therapy via the ETT. Matching PEEP may possibly result in earlier liberation from mechanical ventilation. It should be noted that some clinicians believe that attempts to match PEEP may include the risk that extrinsic PEEP will cause overdistension of the lungs. Overdistension of the lungs may increase the risk of hyperinflation and air leak syndrome. Our studies indicate that spontaneous breathing with pressure support and PEEP lowers WOB. For the individual patient, the level of extrinsic PEEP at which hyperinflation will occur is unknown. Theoretically, for the spontaneously breathing patient, extrinsic PEEP applied to counteract intrinsic PEEP should not cause an increase in end-expiratory lung volume (EELV) until extrinsic PEEP exceeds intrinsic PEEP. Further, the EELV may even decrease, leading to decreased dead space and increased compliance. In our ICU intrinsic, PEEP is measured during a ventilator pause, allowing the patient to exhale completely and measuring the pressure before the next breath. We gradually add extrinsic PEEP with the ventilator and observe RR and clinical work of breathing. We always keep extrinsic PEEP at a level below intrinsic PEEP. We continue to reassess the level of extrinsic and intrinsic PEEP as the patient responds to therapy. Further research is needed to determine the best practice of mechanical ventilation for intubated asthmatics, but this is limited by the small number requiring intubation each year.
Inhalational Anesthetics
One of the properties of inhaled anesthetics is bronchodilation. In turn, inhaled anesthetics have been used as rescue therapy in children with critical asthma who are intubated. We have used isoflurane in our ICU for its reduction in bronchospasm with the added benefit of a decreased sedation requirement. However, inhaled anesthetics are difficult to use in an ICU setting. Modern ICU ventilators are not designed to accept a vaporizer. There is no circle system in ICU ventilators, so there is a tremendous use of anesthetic vapor. There is no primary means of gas scavenging in an ICU ventilator, so significant steps must be taken to contamination of the patient room. There is a case series of six patients reported by Wheeler et al. Dr. J. Tobias has published several articles detailing the use of inhaled anesthetics in asthma and other clinical conditions. A recent retrospective cohort study by Char et al. in intubated asthmatics did not demonstrate a difference in mortality between centers that did or did not use inhaled anesthetics. There was significantly greater length of mechanical ventilation, greater length of stay, and increased hospital charges in the centers using inhaled anesthetics. The significant expertise needed to safely deliver inhaled anesthetics may limit its use to a few centers. An anesthetic conserving device (AnaConDa Sedana Medical) is available in the European market but not the United States. The device is a miniature vaporizer and a conserving medium or reflective filter that keeps the inhaled anesthetic on the patient side of the device. This device can be used with a normal ventilator. Finally, as the scientific community learns more about the potential neurotoxicity with inhaled anesthetics, the physician must weigh the risk and benefit profile to long-term inhalational anesthetics for the management of status asthmaticus.
Extra Corporeal Life Support for Status Asthmaticus
ECLS has been used as a rescue therapy in near fatal asthma. As with inhaled anesthetics, the use of ECLS for this indication is likely limited to a small number of centers. One single center study reports their use, but the number of patients (13 total) is too small to determine whether this therapy has advantages over mechanical ventilation or conventional therapy.
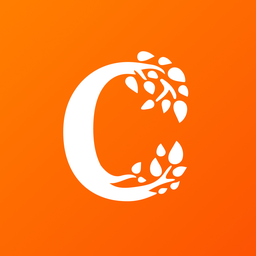
Full access? Get Clinical Tree
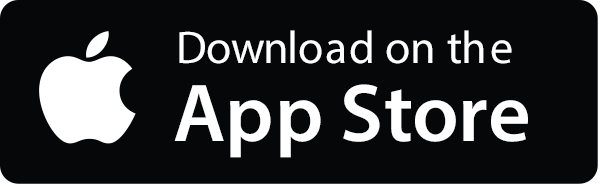
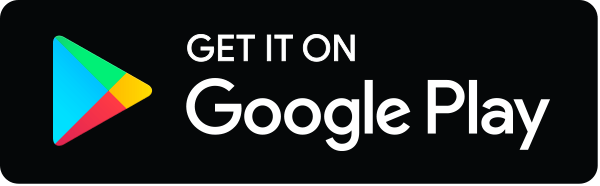