Many xenobiotics adversely affect the senses of olfaction, gustation, and cochlear–vestibular functions. Although these toxic effects are not life-threatening they are frequently distressful to patients. Furthermore, because of the dearth of access to standardized diagnostic techniques and normal parameters, such adverse effects are sometimes overlooked or dismissed by health care providers, despite significant patient distress and dysfunction. This is particularly true for disorders of olfaction and gustation. This chapter reviews the anatomy and physiology of these senses, describes the effects of xenobiotics on these senses, and examines the significant diagnostic information these senses contribute to identifying the presence of xenobiotics. Understanding the effects of xenobiotics on these senses allows for early detection, removal, and prevention of future events. In occupational settings, understanding these principles help prevent permanent and life-threatening injuries.
Olfactory receptors are bipolar neurons located in the superior nasal turbinates and the adjacent septum. There are 10 to 20 million receptor cells per nasal chamber, and the receptor portion of the cell undergoes continuous renewal from the olfactory epithelium.122,127 Renewed olfactory receptors regenerate neural connections to the olfactory bulb. These olfactory receptor neurons are distinctive in their ability to regenerate.26 The axons of these cells form small bundles that traverse the fenestrations of the cribriform plate of the ethmoid bone to the dura. Within the dura, these bundles form connections with the olfactory bulb from which neural projections then connect to the olfactory cortex. There are extensive interconnections to other parts of the brain, such as the hippocampus, thalamus, hypothalamus, and frontal lobe, suggesting effects on other biologic functions.122 Although primary odor detection is a function of the olfactory nerve (CN I), some irritant odors, such as ammonia and acetone, are transmitted through the trigeminal nerve (CN V) and its receptors.48,164
The actual olfactory receptor sites are guanine nucleotide protein (G protein)-coupled receptors (GPCRs) similar to the taste receptors of the mouth and the photoreceptors of the retina. The receptor is a single-polypeptide chain consisting of approximately 350 amino acids, which folds back and forth onto itself to traverse the cellular membrane 7 times. The outer end of the polypeptide contains an amine group (N-terminal), and the cytosolic end contains a carboxyl group (C-terminal). The transmembranous portions determine the receptor shape and characteristics of the binding site. When a molecule binds to a specific receptor site, the resultant conformational change leads to the activation of the G protein system and calcium and/or sodium channel activation and neurotransmission.86
Smelling is an extremely sensitive mechanism of detecting xenobiotics. Olfactory receptors detect the presence of a few molecules of certain xenobiotics with a sensitivity that is superior to some of the most sophisticated laboratory detection instruments, even though human smell is several orders of magnitude less perceptive than other mammals.78
The sense of smell is extremely useful as a toxicologic warning system. Human olfaction is a variable trait.5,137,202 For example, 40% to 45% of people have specific anosmia (inability or loss of smell) for the bitter almond odor of cyanide.49,101,137 There are limited data on the inheritance characteristics or genetic basis of these specific forms of anosmia. Although some studies suggest that the ability to detect the odor of cyanide is a sex-linked recessive trait,68 other studies yield conflicting results.5,20,103 Women have a greater ability to detect androsterone, which is prominent in human underarm secretion.78 Human olfaction usually can distinguish a mixture of no more than 4 xenobiotics;107 therefore, specific odors are frequently masked by other stimuli.
Olfactory fatigue is the process of olfactory adaptation following exposure to a stimulus for a variable period of time. This leads to a temporal diminution of the smell. Unfortunately, this adaptation provides a false sense of security during continued exposure to a xenobiotic. For example, hydrogen sulfide, which inhibits cytochrome oxidase, is readily detectable as distinct and offensive at the very low concentration of 0.025 ppm. At a higher and potentially toxic concentration of 50 ppm, the odor is less offensive, and extinguishes recognition after 2 to 15 minutes of exposure.8,177 At even higher concentrations when toxicity is likely, the onset of olfactory fatigue is even more rapid. The combination of the rapid onset of olfactory fatigue and systemic toxicity at high concentrations of hydrogen sulfide exposure contributes to numerous fatalities (Chap. 123).1,27,185
In industrial settings, it is important to be aware of impaired olfactory function in any worker who may be exposed to chemical vapors or gases.84,177 Such workers are at increased risk for toxic injury. The National Institute for Occupational Safety and Health (NIOSH) requires that an individual using an air-purifying respirator be capable of detecting the odor of a xenobiotic at concentrations below those producing toxicity.6,177 Sensory perception at this concentration ensures that the individual can detect filter cartridge “breakthrough” or failure at a safe concentration.177 The odor safety factor refers to the ratio of the time-weighted average (TWA) threshold limit value (TLV) to the odor threshold for a given xenobiotic. A xenobiotic with a high odor safety factor is detectable despite prolonged exposure.6 Nontoxic xenobiotics with a very high odor safety factor, such as ethyl mercaptan, are added to xenobiotics that are odorless with lower safety factors so that olfactory detection is predictable. This enhanced sensory awareness is the basis for the addition of mercaptans to the odorless natural gases used in the home so as to limit the potential for unrecognized hazardous exposure.
The recognition of odors was traditionally considered an important diagnostic skill in clinical medicine. Some diseases are diagnosed accurately by recognizable associated odors of various affected parts of the body such as the breath, sweat, urine, and wounds: diabetic ketoacidosis as a characteristically fruity odor of the breath; diphtheria as sweet; scurvy as putrid; typhoid fever as fresh-baked brown bread; and scrofula as stale beer.42 Odors are also described for disorders of amino acid and fatty acid metabolism, such as phenylketonuria, maple syrup urine disease, hypermethioninemia, and isovaleric acidemia.42
The recognition of odors continues to be an important diagnostic skill for the rapid detection of some xenobiotics (Table 25–1). To increase awareness of odors of toxic xenobiotics, a “sniffing bar” of commonly available odors may be prepared.73 Nontoxic xenobiotics that simulate the odors of toxic xenobiotics are placed in test tubes, numbered, and inserted in a test tube rack for circulation among staff. The sniffing bar, brief descriptions of clinical presentations, and a table of diagnostic odors (Table 25–1) is used to teach the recognition of odors in medical toxicology.73
Odor | Xenobiotic |
---|---|
Bitter almond | Cyanide |
Carrots | Cicutoxin (water hemlock) |
Disinfectants | Creosote, phenol |
Eggs (rotten) | Carbon disulfide, disulfiram, hydrogen sulfide, mercaptans, N-acetylcysteine |
Fish or raw liver (musty) | Aluminum phosphide, zinc phosphide |
Fruity | Nitrites (amyl, butyl) |
Garlic | Arsenic, dimethyl sulfoxide, organic phosphorus compounds, phosphorus, selenium, tellurium, thallium |
Hay | Phosgene |
Mothballs | Camphor, naphthalene, p-dichlorobenzene |
Pepper | O-Chlorobenzylidene malononitrile |
Rope (burnt) | Marijuana, opium |
Shoe polish | Nitrobenzene |
Sweet fruity | Acetone, chloral hydrate, chloroform, ethanol, isopropanol, lacquer, methylbromide, paraldehyde, trichloroethane |
Tobacco | Nicotine |
Vinegar | Acetic acid |
Violets | Turpentine (metabolites excreted in urine) |
Wintergreen | Methyl salicylate |
There are different types of olfactory dysfunction. Anosmia, the inability to detect odors, and hyposmia, a decrease in the perception of certain odors, are the most common forms of olfactory impairment. The etiology of olfactory impairment is classified as conductive, from anatomic obstruction of inspired air, or perceptive, from dysfunction of the olfactory receptors or signal transmission. Most conductive olfactory dysfunction results in hyposmia, because the obstruction is usually incomplete.122,161
The most common causes of anosmia and hyposmia are viral infections, trauma, xenobiotics, tumors, and congenital and psychiatric disorders (Table 25–2).48,147,153,161 Viral infections impair olfaction either by obstructing nasal airflow or by causing damage to the olfactory epithelium.87 Trauma to the head or nose can shear fragile olfactory nerves crossing the cribriform plate.164,187
Hyposmia/Anosmia | Dysosmia/Cacosmia/Phantosmia |
---|---|
Acrylic acid Antihyperlipidemics: cholestyramine, clofibrate, gemfibrozil, HMG-CoA reductase inhibitors Cadmium Chlorhexidine Cocaine Formaldehyde Gentamicin nose drops Hydrocyanic acid Hydrocarbons (volatile) Hydrogen sulfide Methylbromide Nutritional Vitamin B12 deficiency Zinc deficiency Pentamidine Sulfur dioxide | β-Adrenergic antagonists Amebicides/antihelminthics: metronidazole Anesthetics, local Anticonvulsants: carbamazepine, phenytoin Antihistamines Antihypertensives: angiotensin-converting enzyme inhibitors, diazoxide Antimicrobials Antiinflammatory Antirheumatics: allopurinol, colchicine, gold, D-penicillamine Antiparkinsonians: levodopa, bromocriptine Antithyroid drugs: methimazole, methylthiouracil, propylthiouracil Calcium channel blockers Dimethylsulfoxide Ethacrynic acid Insecticides Lithium Nicotine Nonsteroidal antiinflammatory drugs Opioids Sympathomimetics Toothpastes Vitamin D |
Chronic exposures to numerous xenobiotics are associated with olfactory dysfunction (Table 25–2). The most commonly cited toxic mechanism is perceptive olfactory dysfunction. This is a result of a direct injury, or of a structural alteration of the receptor or its components such as G proteins, adenylate cyclase, or receptor kinase.85,86 Anosmia or hyposmia from hydrocarbons, formaldehyde, cadmium, and chemotherapeutics such as cytarabine results from direct effects on the receptor sites.52,86,91 Local effects on the epithelium and the receptors from antibiotic nose drops leads to temporary anosmia and hyposmia.97,201 Inhaled corticosteroids have local effects on the epithelium, as well as direct effects on both G proteins and adenylate cyclase.88 Cocaine insufflation causes direct local effects such as nasal septum perforations, as well as effects on receptor functions.74,76 Because of the limited local effects of most xenobiotics and the regenerative ability of the olfactory receptor neurons, most xenobiotic-induced olfactory dysfunction is reversible.
Many individuals determined to have anosmia actually have congenital anosmia for select molecules, such as hydrogen cyanide, n-butyl mercaptan, trimethylamine, and isovaleric acid.7,48 Some extreme forms of congenital anosmia are associated with other abnormalities, such as Kallmann syndrome, a hereditary form of anosmia associated with hypogonadotropic hypogonadism in which agenesis of the olfactory bulbs and incomplete development of the hypothalamus are responsible for the anosmia.48,164
Dysosmia or parosmia is the distorted perception of smell (Table 25–2). Subclassifications of dysosmia include the perception of foul smell or cacosmia, or phantosmia the sensation of smell without a stimulus, or such as the sensation of the smell of a burnt or metallic material, torqosmia.161 The etiologies are classified as peripheral or central. Peripheral etiologies include abnormalities of the nose, sinuses, and upper respiratory tract. Central etiologies are related to disorders such as Addison disease, hypothyroidism, temporal lobe epilepsy, psychosis, or pregnancy.48,122,162 How these conditions actually alter the perception of smell is unclear. A number of xenobiotics with similar effects are listed in Table 25–2. Bromocriptine alters dopaminergic transmission and inhibits adenylate cyclase. Levodopa affects the dopaminergic transmission and also chelates zinc, which is important in the maintenance of normal receptor functions.86,88
General evaluation of olfactory function includes a detailed history, focusing on types, duration, and progression of symptoms, recent illnesses, head and nose trauma, sinus diseases, family history, occupational history, exposures hobbies, and xenobiotic exposures.44,77 A physical examination with a detailed examination of the nasopharynx and sinuses is performed to assess the potential for inflammation or structural abnormality. A simple set of olfactory stimulants, such as ground coffee, almond extract, peppermint extract, and musk, is recommended to test each nostril individually with the patient’s eyes closed.77,164 Standardized smell tests such as the University of Pennsylvania Smell Identification Test (UPSIT) and the Connecticut Chemosensory Clinical Research Center (CCCRC) tests are commercially available, and a composite score based on a panel of tests determines the degree of olfactory dysfunction.114 Pungent odors or stimulation associated with ammonia, capsaicin, acetone, and menthol are dependent on the trigeminal nerve (CN V) olfactory function, which is mainly responsible for tactile pressure, pain, and temperature sensation in the mouth and nasal cavity. A patient who has olfactory nerve damage should be able to detect these substances; conversely, a person with hysteria may deny detection of these substances that should physiologically be recognized.77,164,201 If a xenobiotic-mediated mechanism is suspected, the offending xenobiotic should be discontinued. Coronal computed tomography of the sinuses and nose or magnetic resonance imaging of the brain should be obtained if structural abnormalities are suspected.164,201 Gas chromatographic analysis of the urine is useful in patients with fish odor syndrome associated with trimethylaminuria.108,174 Complicated cases and patients with significant impairment should be referred to an otolaryngologist or neurologist.
Taste, the sensory interpretation of oral materials, is determined by taste buds on the tongue, palate, throat, and upper third of the esophagus. The cells in the taste buds have a life span of 10 days and are constantly renewed.11,164 The taste buds on the anterior two-thirds of the tongue and the palate are innervated by the facial nerve (CN VII), those on the posterior one-third of the tongue by the glossopharyngeal nerve (CN IX), and those on the laryngeal and epiglottal regions by the vagus nerve (CN X). The signals are sent to the solitary nucleus in the brain stem, where they are processed and distributed to various regions of the brain. There are at least 14 known chemical taste receptors responsible for the 5 primary taste sensations—sweet, sour, bitter, umami (savory), and salty: 2 sodium receptor types; 2 potassium receptor types; one chloride receptor; one adenosine receptor; 2 inosine receptors; 2 sweet receptor types; 2 bitter receptor types; one glutamate receptor; and one hydrogen ion receptor.79 One substrate will typically activate multiple taste receptors; the combined effects of these stimulated receptors determine the taste of the substance.66
The structure of the taste receptors is similar to that of the olfactory receptors, in that they are coupled to G proteins and sodium and calcium channels permitting neural stimulation. Each receptor is capable of interacting with various classes of xenobiotics, of varying sizes. The pH of the xenobiotic determines sour (acid taste), whereas sodium or potassium concentrations determine salty taste. Many xenobiotics such as sugars, glycols, aldehydes, ketones, amides, amino acids, inorganic salts of lead, and bretylium activate the sweet receptors. Bitter taste results from long-chain organic substances containing nitrogen, or alkaloids, including quinine, strychnine, caffeine, and nicotine.13,79 The threshold for bitter receptor stimulation is several orders of magnitudes lower than other taste receptors.13 Umami taste is a more recently accepted primary taste, best defined as a pleasant savory taste. The primary xenobiotic that stimulates umami receptor is glutamate, such as monosodium-L-glutamate. Umami receptor stimulation is enhanced by 5′-ribonucleotide monophosphates such as inosine and guanosine.205 Salivary proteins, such as zinc containing gustin and ebnerin, are important in the regulation of taste sensation.86,90,110,172 These molecules serve as binding proteins and growth factors for the regeneration of taste receptors. Taste is also affected significantly by the appreciation of aromas or odors and, to a lesser extent, by visual perception.162
Types of gustatory dysfunction include ageusia, the inability to perceive taste; hypogeusia, the diminished sensitivity of taste; and dysgeusia, the distortion of normal taste. There are several variations of dysgeusia, such as cacogeusia, which is a perceived foul, perverted, or metallic taste.77,119 Taste impairment is commonly related to direct damage to the taste receptors, adverse effects on their regeneration, or effects on receptor mechanisms.87 These effects result from a xenobiotic, disease, aging, and nutritional disorders (Table 25–3).72,79,152,183 Any abnormality that interferes with either the direct contact of a xenobiotic with the gustatory cells of the tongue or cranial nerves VII, IX, or X dramatically affects taste.161 Most common forms of xenobiotic-induced dysgeusia are related to direct effects on the taste receptor site or effects related to receptor mechanisms such as G proteins, adenylate cyclase, and calcium channels.106 Other forms of dysgeusia result from direct stimulation of chemical receptors by xenobiotics.79,86
Hypogeusia/Ageusia | Dysgeusia | Metallic Taste |
---|---|---|
Local Chemical and thermal burns Radiation therapy Systemic ACE inhibitors Amiloride Amrinone Carbon monoxide Cocaine Dimethylsulfoxide Gasoline Hydrochlorothiazide Methylthiouracil Nitroglycerin NSAIDs Penicillamine Propranolol Pyrethrins Smoking Spironolactone Triazolam | Local Chemical burn Radiation therapy Systemic ACE inhibitors Adriamycin Amphotericin B Botulism (in recovery) Carbamazepine Dimethylsulfoxide 5-Fluorouracil Griseofulvin Isotretinoin Levodopa Nicotine Nifedipine NSAIDs Phenylthiourea (hereditary) Quinine Zinc deficiency | ACE inhibitors Acetaldehyde Allopurinol Arsenicals Cadmium Ciguatoxin Copper Coprinus spp Dipyridamole Disulfiram Ethambutol Ferrous salts Flurazepam Iodine Lead Levamisole Lithium Mercury Methotrexate Metformin Metoclopramide Metronidazole Pentamidine Pine nuts Procaine penicillin Propafenone Snake venom Tetracycline |
Angiotensin-converting enzyme (ACE) inhibitors commonly cause gustatory impairment, usually hypogeusia and dysgeusia.19,74,121,203 Angiotensin-converting enzyme inhibitors work by inhibiting zinc-dependent ACE, and chelating zinc from taste receptors and salivary proteins results in gustatory dysfunction. Calcium channel blockers act by inhibiting calcium channels of the taste receptor mechanisms.79 Many diuretics cause zinc depletion by enhancing zinc elimination in the urine,79 whereas furosemide and spironolactone may also chelate zinc. Numerous xenobiotics also cause gustatory dysfunction through variable degrees of zinc chelation: amrinone, ethambutol, hydralazine, methyldopa, the nonsteroidal antiinflammatory drugs (NSAIDs), antithyroid medications, penicillamine, and phenytoin.79,86,204 Arsenic, mercury, chromium, and lead either lead to chelation of zinc or replace zinc in salivary proteins because of a higher level of affinity. Chemotherapeutics and colchicine inhibit cellular division and taste receptor regeneration. The oral antiseptic chlorhexidine directly alters taste receptor function.62 Acetazolamide causes cacogeusia when carbonated beverages are consumed. The exact mechanism is unclear, but is postulated to be a result of the inhibition of carbonic anhydrase causing carbon dioxide accumulation and an increased tissue bicarbonate.86,99,124
Normal hearing begins when sound waves are captured by the external auricle, traverse the external auditory canal, and are conducted to the tympanic membrane, the 3 auditory ossicles of the middle ear, and move (although dampened) through the oval window to the perilymph in the scala vestibuli of the cochlea (Figs. 25–1 and 25–2). The sound wave is then transferred through the Reissner membrane at the roof of the cochlear duct, to the endolymph and the organ of Corti.58,178 The specialized hair cells of the organ of Corti convert mechanical waves into neurologic signals. The hair cells contain crosslinked stereocilia projections that detect transmitted shear forces, which lead to the influx of potassium from the endolymph through opened potassium channels.46,112 Depolarization of the hair cells results in calcium influx and neurotransmitter release to the cochlear nerve. Repetitive movements of the stereocilia will amplify the specific sound signals. Neurologic signals from the cochlear nerve are conducted to the cochlear nucleus of the pons; bilateral projections are then sent to the superior olivary nucleus of the midbrain, nuclei of lateral lemnisci, inferior colliculus, medial geniculate body of the thalamus, and then to the auditory cortex of the temporal lobe.178 Interruption or damage to any part of the hearing mechanism will lead to auditory impairment.
The anatomy and physiology of the cochlea and its importance in the biomechanics of hearing are reviewed in order to better understand the potential for xenobiotic injury. The word cochlea is derived from the Greek word kochlias, meaning snail, and describes its general structure—a 2.5-turn, spirally wound tube. The cochlea is further divided into 3 inner tubular structures: the upper tube or scala vestibuli, the middle tube or cochlear duct, and the lower tube or scala tympani. The scala vestibuli and the scala tympani contain the perilymph fluid. The cochlear duct contains endolymph fluid, the Reissner membrane at the roof, and the organ of Corti.178 The cochlear fluids serve multiple functions: to conduct sound waves to the hair cells; to provide nutrients for and remove waste from the cells lining the cochlear duct; to control pressure distribution in the cochlea; and to maintain an electrochemical gradient for the function of the hair cells. The sodium concentration of the perilymph is similar to that of the extracellular fluid, and the potassium concentration of the endolymph is similar to that of the intracellular fluid.60 Any significant alterations of the sodium or potassium concentrations will depress the cochlear potential and function. The stria vascularis controls the production of the cochlear fluids and the repolarization of the hair cells, and maintains the electrochemical gradient between the endolymph and the perilymph. The stria vascularis contains a high concentration of the oxidative enzymes, Na+,K+-ATPase, adenylate cyclase, and carbonic anhydrase, which are all highly susceptible to xenobiotics.21,92,159
Although human speech is composed of sounds in the frequency of 250 to 3,000 Hz, humans can normally detect sounds in the frequency range of 20 to 20,000 Hz.136 The cochlea is a “tuned” structure with varying width and stiffness, such that different regions receive different sound waves. The stiffer and wider base of the cochlea serves as a receptacle for higher-frequency sounds, whereas the apex is responsible for receiving the lower-frequency sounds.58 Because various regions of the cochlea are susceptible to different forms of injury, audiologic testing is tailored specifically to each patient.29
Ototoxicity includes effects on the cochlear and vestibular system. This section focuses on cochlear toxicity. Quinine and salicylates were widely recognized in the 1800s and streptomycin in the 1940s as causes of ototoxicity.92,154 Drugs of abuse such as cocaine and heroin also cause hearing loss.36,40,133,165,167,173,182 Several hundred xenobiotics are implicated as causes of either reversible or irreversible ototoxicity (Table 25–4).23,96,104,130 Ototoxic xenobiotics primarily affect 2 different sites in the cochlea: the organ of Corti—specifically the outer hair cells—and the stria vascularis. Because of the limited regenerative capacity of the sensory hair cells and other supporting cells, when significant cellular damage occurs, the loss is often permanent.50,58,92,176 Although cell death of the outer hair cells from inflammation and necrosis is expected when sufficient insults occur, apoptotic cell death is now postulated to be a major mechanism of ototoxicity from certain xenobiotics such as cisplatin and aminoglycosides.21,23,47,92,96,151 Inhibition of caspases and calpain associated with apoptosis of the hair cells decreases ototoxicity from cisplatin and aminoglycosides in animals.151,175 Heat shock proteins are endogenous molecules that respond to cellular stress and inhibit apoptosis. Xenobiotics, such as celestrol, that can up-regulate heat shock proteins prevent cisplatin and aminoglycoside toxicity in animal models.43,64 Further studies are required to demonstrate clinical utility. Evidence supports the concept that otic injury is potentiated by loud noises. Although the actual cellular mechanisms for many forms of ototoxicity remain unclear,200 some of the mechanisms are known.69 Loop diuretics, such as furosemide, bumetanide, and ethacrynic acid, cause physiologic dysfunction and edema at the stria vascularis, resulting in reversible hearing loss.92,117,194 The underlying mechanisms are the inhibition of potassium pumps and G proteins associated with adenylate cyclase.9 Studies of loop diuretics demonstrate decreased potassium activity in the endolymph and a decreased endocochlear potential.156 Permanent hearing loss associated with furosemide and ethacrynic acid is also reported, and appears to be related to direct interference with oxidative metabolism in the outer hair cells.105,117,166
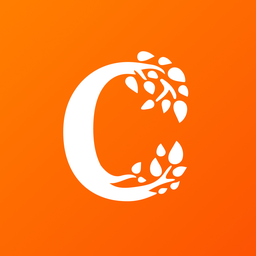
Full access? Get Clinical Tree
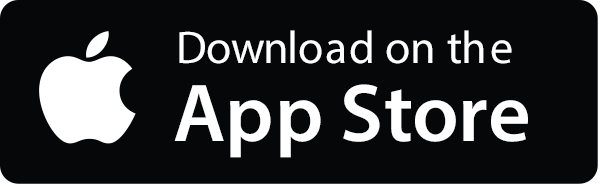
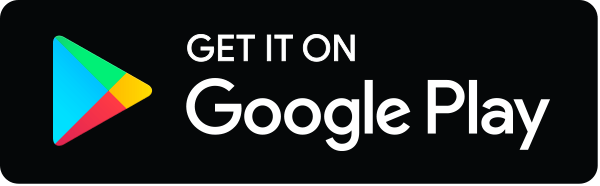