FIGURE 65.1 This 17-year-old girl sustained a high-energy distal tibial fracture as a result of a motorcycle crash. Open reduction with plate fixation offers the best chance of achieving anatomic restoration of articular congruity and reduction of the risk of posttraumatic arthritis.

FIGURE 65.2 The fracture was treated with early intramedullary nail fixation. With early fixation of femoral fractures in multiply injured patients, many pulmonary problems can be avoided. Stable fixation of even complex, comminuted injuries can allow early weight bearing and facilitate functional rehabilitation despite polytrauma.
IM nailing does not typically require direct exposure of the fracture fragment. Theoretically, there is less soft tissue injury and less blood loss associated with IM nailing as compared to ORIF of fractures. However, the fracture fragments and injured muscle remain in situ. Passage of a nail through the medullary canal is also associated with liberation of a number of mediators and/or fat globules into the systemic circulation; these—alone or in combination—can produce systemic inflammatory response syndrome (SIRS) and/or acute lung injury (ALI). In addition, driving the nail through the medullary canal can produce significant bleeding around the fracture itself, which may not be appreciated as it is not directly visualized in the operative field. IM nailing is generally used for most midshaft femur and tibia fractures. It is necessary to have adequate bone on either side of the fracture in order to achieve sufficient fixation to restore stability.
External fixation involves placing an external frame that spans the fracture, limiting fracture movement (Fig. 65.3). External fixation achieves relatively good fracture stabilization, though it may not produce optimal fracture reduction, particularly with periarticular injuries. It can be rapid and noninvasive and result in substantially less blood loss than ORIF or IM nailing. Pins are placed into the bone on either side of the fracture connected to a frame. This can be used as definitive fixation for some fractures if acceptable restoration of length, alignment, and stability has been achieved.

FIGURE 65.3 This patient’s pelvic ring injury was treated with posterior screw fixation and an anterior external fixator.
Open fractures require an initial debridement and irrigation of the fracture fragments as a minimum. This is done to reduce the rate of early soft tissue infection and late osteomyelitis. Although ideal operative therapy of fractures involves irrigation, debridement, and definitive fracture fixation, this is not always the wisest course. Options include irrigation and debridement which can be followed by temporary external fixation. In some cases, irrigation and debridement can be followed by simple splinting of the fractures if there are more immediate patient care priorities. This technique is ideally performed in the operating room and involves debridement followed by pulse lavage. Irrigation and debridement can be performed in the ICU and emergency department if patients are too physiologically unstable to go to the operating room. Patients can be temporized with a bedside procedure if that is all they are able to tolerate initially or if there are other more urgent priorities. Although the data supporting any treatment algorithm are not definitive, most studies suggest that rates of infection are similar provided operative debridement is accomplished within 24 hours of injury (4).
OPTIMAL TIMING OF FRACTURE FIXATION
There are a significant number of advantages to early definitive fracture fixation. Ideally, this should take place as soon as possible following patient presentation. Although the definition of early fracture fixation has varied over the years, most would agree that fracture fixation within 24 hours is considered early. The advantages of early fixation may be most pronounced in patients with multiple system injury. Obviously, these are also the patients most at risk for systemic complications from a significant operative procedure. Thus, experienced judgment must be used in weighing the various risks and benefits to early fracture fixation.
Patients who are initially managed nonoperatively are often treated in some type of balanced traction, particularly for femoral and acetabular fractures. This requires that the patient remain supine in bed. Patients in this position, however, are at risk for developing dependent atelectasis resulting in worsening respiratory failure; these patients may also be at increased risk for aspiration. Patients with traumatic brain injury and intracranial hypertension may have worsening of their intracranial pressures (ICPs) if they cannot have the head of the bed elevated with this positioning. Even though the fracture is somewhat reduced by the traction apparatus or by a splint, as with any fracture, motion at the fracture site worsens bleeding and produces pain and such motion is only reduced by traction and splinting, not eliminated. Either bleeding or pain can increase the systemic inflammatory response. Any immobilized patient is at risk for thromboembolic complications such as deep vein thrombosis or pulmonary embolus, and those in traction may be at particular risk. Patients kept at bed rest in traction cannot be rolled from side to side, producing pressure ulcerations on areas in contact with the bed. Finally, the incidence of fat embolism syndrome (FES) may be higher in patients who undergo delayed fracture repair.
In 1985, Seibel et al. published a prospective nonrandomized cohort study of patients with femoral shaft fractures or acetabulum fractures who also had additional injury (5). All aspects of care of the patients were reportedly standardized except fracture management. The patient population in this study was divided into four groups. The first group underwent immediate (defined as <24 hours after injury) operative fixation of their femoral or acetabular fracture. The second group underwent an average of 10 days of femoral traction. The third group underwent up to 30 days of femoral traction. The fourth group had special circumstances that were predicted to potentially contribute to their pulmonary failure and septic state. Ventilator days and intensive care days were both substantially greater in the groups that underwent prolonged traction than in the immediate fixation group, supporting the conclusion that femoral traction was detrimental in the setting of blunt multiple trauma. This led the authors to propose a number of principles governing the care of patients with multisystem trauma and fractures. They proposed that the fracture hematoma was itself a metabolically active organ, capable of producing multiple organ failure. Early fracture fixation allowed for irrigation of the fracture hematoma. They also included the orthopedic surgeon as one of the frontline members of the trauma team, emphasizing the need for early consultation. In addition, they strongly advocated for fracture repair on the night of admission including using two teams to simultaneously repair fractures if that was available and prudent. They argued that all efforts must be made to support the patient through early fracture fixation as they had demonstrated far better results with this technique as opposed to delaying repair for some days to allow patient stabilization.
Following this report, others also demonstrated better results with early fracture fixation (6–8). Fracture fixation within 24 hours became the standard of care. However, in the early 1990s, data began being published that suggested that not every patient benefited from early fracture fixation. In fact, these studies strongly suggested that patient outcome was much more a function of underlying injury and not so much the timing of fracture fixation. Poole et al. published a series of patients with long bone fracture and traumatic brain injury (9). They were unable to demonstrate any advantage to early fracture fixation, and instead argued that the severity of the brain injury was the ultimate determinate of final outcome. Rogers et al. addressed the cost of performing early fracture fixation, particularly on off hours in hospitals that did not have in-house operating room resources (10). Outcomes were the same when femur fractures were repaired in the middle of the night as compared to a group of patients who were placed on the operating room schedule for the next day; the cost, however, was significantly higher in patients who underwent emergency fracture fixation.
It is unclear as to why these authors were unable to substantiate the findings that seemed to show clear benefit to early operative fixation. One possibility is that the care during the critical care phase of these multiply injured patients improved over the late 1980s and early 1990s. End points of resuscitation were defined (11) and the role of invasive monitoring was clarified (12). Specific needs for patient populations such as geriatric trauma patients were defined (13). Thus, it is possible that the time taken to stabilize the patient in the ICU preoperatively was now, in fact, time well spent.
In the mid- to late 1990s, several papers were published that strongly suggested that early fracture fixation was, in fact, dangerous, particularly in patients who sustained traumatic brain injury. The group from Yale reported on 32 patients treated over a 5-year period with brain injury and long bone fractures (14). Those who underwent early fracture fixation, defined as within the first 24 hours of admission, had statistically significant worse neurologic outcome at the time of discharge. This was thought to be secondary to the increased rate of intraoperative hypotension and hypoxia seen in patients with early fracture fixation. Townsend et al. observed similar findings (15). In their study, episodes of intracranial hypertension were more common in patients who underwent early fracture fixation. They also noted an increased rate of intraoperative hypotension and hypoxia; this risk seemed to persist for approximately 24 hours.
Scalea et al., however, reported different findings. They studied over 180 patients with long bone fracture and traumatic brain injury over the same 5-year period as studied by the group at Yale (16). They found no difference in neurologic outcome in the group of patients treated with early fracture fixation.
How, then, should a critical care physician decide when a patient ought to have fracture fixation? It would seem reasonable to address a number of issues. The first would be to assess the risk of anesthesia and the magnitude of the operative procedure. There certainly are scoring systems that can be applied to assess perioperative risk; careful clinical assessment may be equally good. The second issue would be to examine operative options. For instance, does the patient have a reasonable nonoperative option? Would a lesser procedure, while perhaps not ideal, at least be adequate? This obviously would require a discussion between the critical care service and the orthopedic surgeons, trauma team, and anesthesiologist. Finally, one must ask whether the patient is in optimal condition. Can we reduce perioperative risk with a reasonable period of preoperative optimization? Can cardiac performance be improved with volume and/or inotropes? Will 1 to 2 days allow ventilatory requirements to be reduced? Would a delay improve renal function? Only when all of these questions are answered can an intelligent decision about the optimal time and fracture fixation be made.
DAMAGE CONTROL ORTHOPEDICS
Damage control is a technique developed in the late 1980s and early 1990s in early urban American trauma centers. This shift in approach coincided with a period of time when rates of penetrating trauma were increasing. Not only was penetrating trauma more common, but also multiple high-velocity missiles became the norm, rather than the exception. Traditional teaching had been to repair all injuries at the time of initial surgical procedure. Unfortunately, this often required prolonged operative care. In the operating room undergoing lengthy operative procedures, patients developed what was termed the lethal triad of acidosis, coagulopathy, and hypothermia, only to arrive in the ICU and die of acute organ failure, usually 6 to 24 hours postoperatively (Table 65.1).
Many trauma centers began using a staged approach with encouraging results. In 1993, Rotondo et al. published the series that named the technique “damage control,” and demonstrated statistically significant better outcomes in a group of patients treated with the staged care as opposed to definitive care at the index operation (17). When damage control is used for abdominal injuries, only life-saving procedures are performed at the time of the initial procedure. Important but non–life-threatening injuries such as gastrointestinal injuries are simply temporized. Nonsurgical bleeding is controlled with packing and the fascia left open to avoid the potential of developing abdominal compartment syndrome. A vacuum dressing, either one that is commercially available or homemade is often used. Some data exist suggesting the type of dressing used may affect the degree of inflammation stemming from the abdominal cavity (18). The patient is then admitted to the ICU and resuscitated. When physiologically stable, the patient can be brought back to the operating room for re-exploration, gastrointestinal reconstruction, and an attempt at fascial closure. The same principles can be applied to bony injuries. Fractures are common and occur in over 75% of multiply injured patients. There are clear advantages to early fracture fixation. Yet, some patients may not be best served by such technique. A technique that would achieve many of the advantages of fracture fixation without the disadvantages of definitive surgery would be advantageous for some patients.
Remote organ dysfunction occurs not only in association with, but also as a direct consequence of, long bone fractures. In addition to the fracture itself, soft tissue injury, compartment syndrome, infection, and extremity ischemia-reperfusion injury can all be associated with release of toxic mediators that can cause remote endothelial cell damage. The primary target of this remote organ injury appears to be the lungs, but secondary targets include the gut, kidney, and brain. The resultant injury is progressive and can lead to multiple organ dysfunction syndrome (MODS). This understanding led to the development of the concept of damage control orthopedics (DCO). DCO is the process by which temporary stabilization of long bone fractures is accomplished using techniques not associated with secondary systemic injury. Secondary definitive stabilization is delayed until after physiologic stabilization has been achieved (Fig. 65.4 to 65.6). The goal of damage control therefore is to provide the patient with the benefits of early stabilization of long bones without exposing him or her to the risks associated with definitive stabilization procedures.
TABLE 65.1 Principles of Damage Control |
![]() |
Several groups of surgeons have engaged in the process of temporary stabilization of long bone fractures in polytrauma patients using techniques associated with minimal systemic consequences. Scalea et al. published their experience with external fixation as a temporary tool for stabilization of long bone fractures in polytrauma patients in 2000 and coined the now commonly used term DCO (19). They concluded that external fixation was an alternative mechanism of achieving temporary stabilization in multiply injured patients and that it was rapid, associated with minimal blood loss, and safely convertible to better definitive fixation after appropriate delayed physiologic stabilization of the patient. Other authors have expanded on the DCO concept and have reported more specifically on its efficacy (20).
The physiologic rationale for DCO is based upon the timing and extent of the initial inflammatory response that follows a major injury. In most individuals, that initial inflammatory response is followed by a counterregulatory anti-inflammatory response that leads to spontaneous recovery. In situations where the initial inflammatory response is excessive, secondary remote organ injury such as ALI related to increased pulmonary capillary membrane permeability can occur. The likelihood of this secondary remote organ injury developing may be increased in situations associated with substantial ischemia-reperfusion injury—such as prolonged shock—continued loss of body temperature, tourniquet-mediated ischemia-reperfusion injury, surgical blood loss with failure of resuscitation, and embolization of fat and marrow contents secondary to instrumentation of the femoral or tibial canal. This secondary remote organ injury is characterized by increased capillary membrane permeability, ALI, gut bacterial translocation, and acute tubular necrosis. It can be mediated by cytokines, complement, activated neutrophils, eicosanoids, and reactive oxygen products (21). Thus, both early total care and ongoing long bone instability can serve as second hits that lead to the development of severe secondary remote organ injury. In the “at-risk” patient population, early DCO can limit ongoing release of inflammatory mediators and allow for further physiologic stabilization prior to proceeding with definitive stabilization (22).

FIGURE 65.4 Damage control orthopedics case example. This 27-year-old motorcyclist sustained multiple injuries including an open right femoral shaft fracture. He presented in physiologic extremis. Laparotomy demonstrated a grade 5 hepatic injury and a retrohepatic caval injury. Right hepatic lobectomy and repair of a vena caval laceration were performed as part of a damage control laparotomy procedure.

FIGURE 65.5 Damage control orthopedics consisted of rapid debridement of the open femoral fracture plus temporizing external fixation to achieve restoration of femoral length and alignment and decrease ongoing injury secondary to release of inflammatory mediators.

FIGURE 65.6 On postinjury day 5, after adequate restoration of physiologic stability, the patient underwent removal of the temporary external fixator and definitive fixation of the femoral fracture using a reamed intramedullary nail. He was discharged to a rehabilitation facility 19 days after injury.
The issues that led to the use of this damage control strategy have been debated for some time. In 1989, Bone et al. published a prospective randomized trial of early (<24 hours after injury) versus late (>72 hours after injury) nail fixation of femoral shaft fractures (23). They found that in patients with an overall Injury Severity Score (ISS) of greater than 18 (indicative of polytrauma), intensive care days and pulmonary complications were reduced with early fixation as compared to late fixation. They concluded that stabilization of femoral fractures within 24 hours of injury in multiply injured patients decreased pulmonary morbidity as compared to stabilization after 72 hours. The study was flawed in that it was not blinded, that parameters of resuscitation were not defined, and that very few patients with severe pulmonary injury were included in the early fixation group.
In 1993, Pape et al. published a worrisome report implicating primary nail fixation of reamed femoral shaft fractures as a potential cause of posttraumatic acute respiratory distress syndrome (ARDS) (24). They reviewed patients admitted over a period of 10 years with an ISS greater than 18 and midshaft femoral fractures. Those with and without chest injury and those who underwent femoral nailing less than 24 hours and greater than 24 hours after injury were compared. They found a higher incidence of ARDS and death in patients with severe chest trauma who were treated with early reamed nailing as compared to those with severe chest trauma treated with delayed reamed nailing. They concluded that early reamed nail stabilization of femoral shaft fractures in “borderline” patients may increase the risk of secondary pulmonary injury and development of ARDS. They recommended that definitive fracture fixation be delayed in these patients and that temporizing techniques such as external fixation be employed. The criticism of Pape’s study was that the early nailing group included eight patients with pulmonary contusions as compared to only two in the late nailing group. Both Pape et al. (24) and Bone et al. (23) failed to report on any parameters of resuscitation within their patient populations.
In a prospective randomized trial, Pape et al. compared nailing to DCO with regard to immunoinflammatory parameters in patients with tibial or femoral shaft fractures, and ISS greater than 16 (25). Patients with a thoracic abbreviated injury scale (AIS) score greater than 3, ongoing shock, or elevated ICP were excluded. They found that primary femoral nailing was associated with significantly higher systemic interleukin (IL)-6 and IL-8 levels for 48 hours postoperatively compared to either initial DCO procedures or secondary femoral nailing after initial damage control. This suggested that the secondary release of inflammatory mediators associated with femoral nailing can be effectively mitigated by initial DCO with delayed femoral nailing. O’Toole et al. looked at the effect of resuscitation prior to femoral nailing on the development of ARDS in multiply injured patients (26). They utilized a resuscitation protocol that included normalization of lactate prior to IM nailing, resulting in rare need of DCO. The rate of ARDS was also quite low, even in polytrauma patients with serious chest injury.
For that subset of patients whose physiologic condition precludes primary definitive operative stabilization of fractures (early total care), external fixation is a useful technique for temporary stabilization of long bone fractures. Such external fixation is advantageous because it can be applied rapidly, with minimal blood loss, and with sufficient restoration of stability to prevent ongoing soft tissue injury and to prevent ongoing release of inflammatory mediators. Effective limitation of secondary effects of shock has been demonstrated with this type of initial DCO approach (27).
The disadvantages to employing DCO in all patients with even questionable polytrauma are at least twofold. First, employing temporizing external fixation techniques necessitates a return to the operating room for delayed definitive fixation, thus exposing the patient to two separate operative interventions/anesthetics. This is obviously warranted if the benefit is prevention of a serious secondary consequence such as ARDS. In the absence of a clear decrease in the risk of ARDS, it is harder to justify exposing polytrauma patients to a need for secondary surgical procedures. A second major disadvantage to external fixation (a temporary device) is the risk of complications with conversion from external fixation to IM nailing or other definitive treatment. Experience with tibial fractures has suggested that if external fixation is in place for less than 2 weeks, the risk of infection with definitive fixation is lower, particularly if there is no history of pin tract infection at any point (28). In the femur, conversion from external fixation to definitive nailing appears safe within the first 4 weeks following application of the initial frame (29).
Hildebrand et al. evaluated the association between the timing of secondary definitive surgical intervention, inflammatory changes, and systemic outcome (30). They reviewed a prospective cohort of patients treated with DCO. They compared those treated with early secondary surgery (2 to 4 days after initial injury) to those treated with late secondary surgery (5 to 8 days after initial injury). They found that early secondary surgery was associated with a higher incidence of organ dysfunction and concluded that there was no particular advantage to early secondary surgery. They recommended that if DCO is selected, secondary surgery should be delayed more than 5 days after the initial DCO procedure.
The operative procedure of IM stabilization of long bone fractures, both femoral and tibial shaft fractures, is associated with some component of pulmonary injury (31,32). Although fracture fixation–associated pulmonary injury appeared to be well tolerated in the majority of patients, including those with polytrauma, certain susceptible polytrauma patients seemed to be at risk for worsening lung injury. Paradoxically, these are likely the same patients who are at greatest risk for developing secondary pulmonary injury from a nonoperative management of their long bone fractures and the most in need of early fracture stabilization.
DCO, the practice of utilizing temporary external fixation to limit ongoing injury secondary to long bone fractures in situations where definitive operative stabilization is contraindicated, may be effective in patients with polytrauma and lung or brain injury. For the majority of patients with femoral shaft fractures, including most polytrauma patients, primary reamed femoral nailing within 24 hours of injury remains the gold standard of treatment (31). Defining the “at-risk” patients who are unable to tolerate early reamed nailing is difficult, but if adequate resuscitation and normalization of serum lactate is achieved, damage control should rarely be necessary (26). In borderline patients who are physiologically unstable because of severe chest or head injury or inadequate resuscitation, temporizing external fixation and DCO may be advantageous.
FRACTURES IN GERIATRIC PATIENTS
As the US population ages, there is an increasing volume of injured geriatric patients. Injury is now the seventh leading cause of death for those over age 64 (33). Geriatric trauma is increasing both in absolute number and as a proportion of volume presenting to trauma centers each year. Based upon the National Trauma Data Bank, the proportion of trauma patients aged 65 years or older in Level I and II trauma centers increased from 23% in 2003 to 30% in 2009. These numbers are likely underestimates as many injured geriatric patients are treated at non-trauma centers and community hospitals (34,35). There are a number of unique considerations for this patient population that are exceptionally important for those caring for the geriatric patient with orthopedic injuries to recognize.
Elderly patients sustain all the same injuries as the younger trauma patient. Unfortunately, aging is associated with a decrease in the physiologic reserve that many younger trauma patients have. Under normal circumstances, the elderly compensate to meet their needs. However, during times of increased physiologic demand many elderly are unable to compensate effectively. Additionally, pre-existing medical conditions in the elderly limit their ability to tolerate the increased physiologic demands associated with acute trauma. It has been demonstrated that elderly patients have substantially worse outcomes than younger trauma patients (36–38). The cause of this is clearly multifactorial; in addition to decreased physiologic reserve, referred to as fragility (39), comorbid medical conditions, and poorer baseline functional status, osteopenia, and sarcopenia may result in a higher severity of injury (40).
As a general rule, the aim of treatment of geriatric fractures is to obtain a fixation that both reduces pain and permits early mobilization (41). The decision for surgical intervention should be based primarily on the injury pattern and not solely on the age of the patient (42), but it is well recognized that in addition to increased risk of mortality and major morbidity following trauma, geriatric patients also may be at risk of orthopedic-specific complications following orthopedic injuries. Some have demonstrated that advanced age is associated with poor fracture healing due to an increased risk of nonunion due to an inability to achieve mechanical stability in osteopenic bone and overall poorer fracture biology (41,43). However, others have demonstrated that patient age alone at the time of surgery is not independently associated with poorer nonunion of fractures (44,45).
When managing the geriatric patient with orthopedic injuries, although operative fixation is often preferred for many fractures, the risks of perioperative morbidity and mortality from comorbid conditions must be carefully weighed against the benefit of operative stabilization. Careful perioperative risk assessment is appropriate in which the patient undergoes a risk stratification that weighs the patient’s operative risk against the benefit of operative intervention. Patients may be stratified into low, medium, or high risk of events based on history, baseline exercise tolerance, and the absence or presence of active cardiac conditions as well as EKG and echocardiographic findings. This risk can then be balanced against the magnitude of the procedure—high-risk procedures such as large blood loss procedures or complex revisional joint replacement, moderate-risk procedures such as femoral ORIF, and low-risk procedures such as simple upper extremity fracture fixation—and the emergent (such as compartment syndrome or an acutely threatened limb) or urgent (acetabular fracture with an unstable joint or ankle/talus fracture with threatened skin) nature of the procedure.
Although elective surgery in the elderly provides an opportunity to optimize health preoperatively, those having emergency orthopedic procedures are not afforded time for optimization, and may present malnourished, dehydrated, and acutely ill. In geriatric polytrauma patients, this becomes a particular concern as these patients may have competing priorities of care and often will require large volume resuscitation with the goal of oxygen delivery to balance tissue oxygen demand.
Pre- and postoperative monitoring of geriatric patients with orthopedic injuries is essential, although there is some controversy about the optimal way to accomplish this. Fluid management, for example, can be challenging in this patient population. Underlying cardiac and renal dysfunction predisposes elderly patients to volume overload and pulmonary edema but this must be balanced against the need to maintain adequate organ perfusion. Scalea et al. demonstrated significant hemodynamic compromise in elderly patients, clinically stable after initial trauma evaluation, using invasive monitoring (13). The authors demonstrated an increase in survival from 7% to 53% with the use of early optimization of patients with volume, inotropes, and afterload reduction. The authors concluded that emergent invasive monitoring identifies occult shock early and improves outcome. Since this early work, demonstrating the benefit of aggressive and invasive monitoring in geriatric trauma patients, newer monitoring devices including continuous central venous oximetry (ScvO2) and stroke volume variance (SVV) have been introduced that may be used to help guide resuscitation in the elderly trauma patient. Noninvasive hemodynamic monitoring in the elderly, high-risk surgical patient has been shown to be as effective as traditional invasive thermodilution techniques using a pulmonary artery catheter (46,47). Additionally, transthoracic (TTE) or transesophageal echocardiography (TEE) may provide important information regarding volume status and cardiac function to help guide resuscitation.
In addition to the unique challenges facing providers caring for geriatric patients with orthopedic injuries related to poor outcomes due to increased morbidity and mortality, there are also a few important types of fractures relatively unique to this population.
Fractures of the proximal humerus are very common in geriatric population and remain a difficult problem; when associated with rotator cuff injury these can lead to poor functional outcome (41). Fractures of the distal radius are exceptionally common in elderly females and, while most fractures of the distal radius can be treated conservatively, unstable distal radius fractures require surgical fixation. Spine fractures are also quite common in geriatric patients, ranging from vertebral compression fractures that can result in kyphosis to devastating spinal cord injuries, associated with significant mortality (48).
Geriatric hip fractures are also exceptionally common and, whether treated in a trauma center or community hospital, present a significant source of both short-term and long-term pain and disability. Additionally, in elderly patients with hip fractures, mortality of up to 10% at 1 month, 20% at 4 months, and 30% at 1 year are seen (49). Optimal management of these patients is somewhat debated, but two large systematic reviews of thousands of patients have concluded that early surgery—typically within 48 hours of admission—after a hip fracture reduces hospital stay, complications, and mortality (50,51). Optimizing these patients preoperatively can be difficult, however, as many have significant comorbidities. As hip repair surgery is largely considered an intermediate-risk surgery, there is little preoperative intervention that should be performed at the risk of delaying intervention. Medical optimization is appropriate for uncontrolled hypertension, dysrhythmias, and active myocardial ischemia. However, these interventions should not delay surgery beyond 24 to 48 hours given the known risks of increased complication rates in patients in whom surgery is delayed (52).
COMPARTMENT SYNDROME
Compartment syndrome occurs when there is increased tissue pressure within a confined osseofascial space. This increased pressure compromises blood flow and subsequently results in tissue damage if left untreated. The fascia of muscle compartments is stiff and does not expand to accommodate significant swelling. Increases in compartment volume and subsequent pressure can be caused by fractures, soft tissue crush injury, hemorrhage, reperfusion following arterial revascularization, and increased capillary permeability as may occur in the setting of burns or shock states.
Compartment syndrome was first recognized as a causative factor in ischemia of the hand by Richard von Volkmann in 1881 (53). Other investigators subsequently described and confirmed that ischemia of muscle in a fascial compartment was caused by an increase in pressure from compromised venous outflow and edema secondary to reperfusion (54,55). Although most typically found in the leg, compartment syndrome of the arm and hand is well recognized, as is compartment syndrome of the buttock, thigh, and foot (56–59). The reported incidence of compartment syndrome varies widely. Rates of 6% in patients with open tibial fractures and 1% in patients with closed fractures have been reported, but much higher percentages have been published in association with concomitant vascular injury (60,61). In children, rates of up to 20% have been reported with open forearm fractures (56).
Any increase in compartment volume elevates compartment pressures, which, in turn, compromises lymphatic and venous outflow; this leads to additional edema and ultimately arterial insufficiency. Compromised arterial inflow causes tissue ischemia and cellular edema, which only serves to increase compartment pressures further (62,63). Experimental studies have shown that the longer pressure remains elevated, the more severe the muscle and nerve damage (63). Additionally, episodes of hypotension increase the extent of muscle ischemia.
External restriction of an extremity may also lead to compartment syndrome. Compartment syndrome has been described as a result of constrictive casting or dressings and with the use of pneumatic antishock garments (military antishock trousers) (64). Regardless of the underlying cause, if left untreated, the end result of compartment syndrome is muscle and nerve injury from infarction and necrosis.
The clinical diagnosis of compartment syndrome can be elusive, as symptoms may often be ascribed to other causes (65,66). In the alert patient, pain is typically the first symptom. Pain is typically worsened with palpation of the affected compartment and ameliorated with passive stretch of the muscle group. Pain or physical findings are frequently attributed to the associated fracture, however. Sensory deficits may occur late and are typically in the distribution of the sensory nerve traversing the affected compartment. The classic description of sensory loss is in the first web space of the foot secondary to elevated anterior leg compartment pressures and the effect on the deep peroneal nerve. Motor weakness is a late and ominous sign. Loss of palpable pulses is typically associated with vascular injury and is a very late and rare finding in the extremity with compartment syndrome.
In the patient who is not awake and alert, the diagnosis of compartment syndrome requires extreme vigilance and a high degree of clinical suspicion. The diagnosis in these patients requires frequent physical examination and tissue pressure measurements. Tissue pressure measurements are typically accomplished via accessing the affected compartment with a needle or catheter and recording pressure measurements via manometry or pressure transduction. Many techniques have been described, but the simplest and most widely used is the STIC Device (Stryker Corporation, Kalamazoo, MI). The handheld STIC catheter utilizes a disposable syringe and needle and, after zeroing the monitor, insertion of the needle through the fascia of the compartments. It allows for rapid and reliable assessment of compartment pressures (67). Alternatively, a 16-gauge needle attached to a transduction system also can produce reliable measurements (68). Some have advocated continuous pressure monitoring using systems designed specifically for that purpose, although recent data do not support the use of continuous monitoring in the alert patient (69,70). Several researchers have attempted to develop noninvasive methods of compartment pressure measurements with limited success in the setting of acute compartment syndrome (71,72).
There is some debate in the literature concerning the measured pressure at which the diagnosis of compartment syndrome should be made and fasciotomy performed. Many advocate the use of absolute values of greater than 30 to 35 mmHg as an indication for fasciotomy (69,73). Others have stressed the importance of the difference between systemic diastolic pressure and measured compartment pressure (74,75). This difference in pressures has been shown to be a more reliable indicator of impending compartment syndrome. A ∆p of less than 30 mmHg is used by many as an indication for fasciotomy. This highlights the concern that patients who are hypotensive are at significantly greater risk of compartment syndrome than normotensive patients with comparable absolute compartment pressure measurements. Therefore, intraoperative ∆p should be calculated based on the preoperative diastolic blood pressure and compartment pressures should be measured early in the postoperative period (75).
In the patient who is at risk of compartment syndrome, there are some steps that can be taken that may minimize the development of compartment syndrome. First, tight casts, splints, and dressings should be avoided and promptly removed if the patient complains of pain out of proportion to the underlying injury or fracture. There is some controversy about the optimal extremity position in patients at risk for compartment syndrome. Elevation of the extremity may lead to a reduction of arterial perfusion pressure and subsequent blood flow (52,76). Alternatively, placing the limb in a dependent position may exacerbate edema and reduce venous outflow. The optimal position for the patient at risk of compartment syndrome is to place the affected extremity at the level of the heart to optimize both arterial and venous flow.
Surgical decompression is the treatment of compartment syndrome. Care must be taken to ensure that all affected osseofascial compartments are widely opened. The fasciotomy wounds should be left open, as any attempt to provide tissue coverage over the at-risk muscle may lead to additional muscle injury. A variety of techniques have been described for fasciotomy of the various compartments of the extremities.
In the patient at high risk for the development of compartment syndrome, prophylactic fasciotomy may be appropriate. If the patient will be unexaminable, unable to complain of symptoms or unavailable for serial or continuous measurements of compartment pressures, prophylactic fasciotomy may be indicated to prevent the morbid sequelae of untreated compartment syndrome. In patients with prolonged hypotension or arterial vascular repairs, prophylactic fasciotomy should be performed if the affected extremity was poorly vascularized or unvascularized for more than a few hours. In the setting of concomitant venous injury, fasciotomy should not be delayed because venous insufficiency may exaggerate edema and increase the risk of the development of compartment syndrome.
Once fasciotomies are performed, sterile dressings or a closed vacuum suction device should be applied and the wounds re-examined in about 48 hours. If fasciotomy was done late in the course of compartment syndrome, frankly necrotic muscle should be debrided at the time of decompression. Muscle with borderline or questionable viability should be left and re-examined in 24 to 48 hours. Patients who develop hyperkalemia or signs of sepsis early after fasciotomy should be re-explored in the OR to examine muscle viability. Extensive and potentially functionally unacceptable debridement and/or amputation be necessary to salvage life over limb in a patient who is critically ill.
There are a number of techniques available for delayed muscle coverage following fasciotomy that have been described. Closure by secondary intention is an option, as is split-thickness skin grafting. Other techniques of delayed coverage include the “Op-Site roller,” the “shoelace technique,” the STAR (suture tension adjustment reel) method, and vacuum-assisted closure (77–80).
Complications of untreated compartment syndrome include muscle necrosis, irreparable nerve damage, and limb loss (81,82). Delay in therapy may also result in these morbid sequelae (74,81,82). When irreversible ischemia in an affected extremity occurs, there is a depreciable decrease in functional recovery (83,84). Additionally, delay in fasciotomy causes an increase in infection rates, which may lead to sepsis and multiple organ system failure (81,84). When treatment is delayed more than 12 hours, amputation rates may be over 20%.
Massive necrosis of muscle also has significant systemic effects. Myonecrosis can result in the release of large amounts of myoglobin. Myoglobin is also released from damaged muscle, particularly during the reperfusion phase following fasciotomy, causing rhabdomyolysis. Myoglobin can precipitate renal failure via three mechanisms: decreased renal perfusion, renal tubular obstruction due to cast formation, and direct toxic effects of myoglobin on the kidney (85,86). The incidence of renal failure in the setting of rhabdomyolysis is estimated at 4% to 33% and carries a mortality of 3% to 50% (86,87). Early diagnosis is critical to prevention of renal failure, and all patients who have compartment syndrome should be monitored with serial creatine kinase (CK) levels. Generally, CK levels of greater than 5,000 U/L are diagnostic of rhabdomyolysis, although renal failure is usually seen at higher levels (87). The mainstay of treatment of rhabdomyolysis is aggressive hydration and maintenance of high-volume urine output. Some have advocated the use of mannitol and urine alkalinization with bicarbonate-containing solutions, although others have demonstrated no benefit of these therapies over high-volume normal saline hydration alone. Continuous renal replacement therapies can also be used to clear myoglobin from the blood and are advantageous if renal failure occurs due to greater hemodynamic stability and tolerance in critically ill patients.
In addition to poor long-term functional recovery following late or untreated compartment syndrome, a Volkmann contracture may occur. A Volkmann contracture is the chronic limb deformity arising from untreated compartment syndrome causing muscular ischemia and subsequent fibroblastic proliferation, contraction, and adhesion formation. Additionally, nerve ischemia leads to loss of denervation of the muscle, muscle paresis, and paralysis. A Volkmann contracture causes significant long-term morbidity and may lead to a need for amputation or extensive reconstruction to regain function of the affected extremity.
FAT EMBOLISM SYNDROME
FES has been reported to occur in bone marrow transplant, pancreatitis, fatty liver, and liposuction (88,89); however, FES is most commonly associated with long bone fractures. Although fat embolism may occur in up to 90% of trauma patients, FES occurs in only 2% to 5% of patients with long bone fractures (90,91). FES is characterized by both pulmonary and systemic fat embolism and includes a spectrum from subclinical to mild and fulminate presentations (89,92,93). Clinical FES typically involves multiple organ systems; however, involvement of the pulmonary, neurologic, hematologic, and dermatologic systems is the most common.
Fat embolization can occur at the time of fracture. Long bone fixation may result in additional embolization and FES. Elevated pressures during reaming of the IM canal appear to be temporally associated with embolization to the pulmonary circulation when studied with echocardiography (94). Once fat is liberated into the circulation and embolizes, the pulmonary microvasculature becomes occluded. Depending on the size of fat globules, smaller globules may traverse the pulmonary microvasculature and reach the systemic circulation, leading to the common neurologic manifestation of FES. Although the pulmonary, cerebral, retinal, and skin microcirculations are typical clinical manifestations of FES, fat embolization can affect any microcirculatory bed.
ALI and ARDS may result from fat emboli occluding pulmonary capillaries, and biochemical alterations directly damage the pulmonary capillary endothelium (89,95–97). Although many patients with long bone fractures develop fat embolism, far fewer develop FES, suggesting that additional factors may be necessary in the development of lung injury. Biochemical fat embolization is associated with the release of free fatty acids (FFAs) (98). FFAs in the lung are locally hydrolyzed in pulmonary circulation by lipoprotein lipase, which releases toxic substances that injure the capillary endothelium. The release of FFAs increases vascular permeability, producing alveolar hemorrhage, edema, and inactivation of the surfactant molecules (99,100). Ultimately, these pulmonary alterations lead to ALI and ARDS. As fat accumulates in the pulmonary microcirculation and lipoprotein lipase liberates FFAs, disseminated intravascular coagulation (DIC) and platelet aggregation further compound capillary disruption and systemic inflammation.
Fat emboli that pass through the pulmonary vasculature result in systemic embolization, most commonly in the brain and kidneys (101). Cerebral FES is a rare, yet potentially lethal, complication of long bone fractures. Neurologic symptoms vary from confusion to encephalopathy with coma and seizures. A clinical diagnosis may be difficult as cerebral FES may be masked by other clinical scenarios (102). Diffuse encephalopathy, petechial hemorrhages, localized cerebral edema, and white matter changes have also been seen in patients diagnosed with FES. Magnetic resonance imaging (MRI) may show the characteristic cerebral lesions of the acute state of FES as opposed to a computed tomography (CT) scan, which often may appear normal (103).
A specific treatment for FES does not currently exist. Treatments with heparin, dextran, and corticosteroids have not been shown to reduce the morbidity or mortality (88,104). However, when given prophylactically, corticosteroids (methylprednisolone) may have beneficial effects (105,106). The mainstay of treatment for FES is supportive; therefore, prevention, early diagnosis, and adequate symptom management are paramount. Although long bone fracture fixation is the main cause of fat embolism and FES, early fracture fixation may be critical in reducing recurrent liberation of fat into the circulation as a result of fracture movement and decreases the incidence of FES (107).
Patients with polytrauma are at risk of other forms of respiratory failure (atelectasis, pneumonia) and multiple system organ failure (MSOF). Early fixation and patient mobilization may reduce those complications (108). Methods to reduce IM pressure and embolization during reaming have been developed, which include venting or applying a vacuum during reaming to limit the elevation of IM pressure and thus reduce the incidence of fat embolization (109,110).
Respiratory failure from FES is characterized as permeability edema with decreased compliance similar to oleic acid lung injury. Gas exchange abnormalities include shunt and increased dead space from atelectasis and alveolar flooding comparable to ALI and ARDS from other causes (111,112). The general goals of ALI and ARDS management focus on maintaining acceptable gas exchange while limiting ventilator-associated lung injury (VALI).
Patients with FES may develop cerebral edema, leading to rapid deterioration (113). In such cases, ICP monitoring may be beneficial (114). In general, trauma patients should not have their neurologic examination obscured by excessive sedation or neuromuscular blocking agents in order to allow them to tolerate mechanical ventilation (115).
The outcome in patients with FES who receive supportive care is generally favorable, with mortality rates of less than 10% (116). Pulmonary, neurologic, and retinal abnormalities generally resolve completely. General management is supportive in nature and focuses on early fixation and mobilization. Organ support includes shock resuscitation and gas exchange support, which balances lung recruitment and limits the potential for VALI. Ideally, neurologic support would include the ability to conduct a clinical neurologic examination.
PELVIC FRACTURES
Pelvic fractures occur from high impact trauma and are usually associated with mortality rates ranging from 10% to 15% (117). Initial care of patients with suspected pelvic fractures is not different from any other trauma patient. Signs of hemorrhage must be identified and resuscitation initiated; as with other patients, limiting crystalloid is wise. Blood, plasma, and platelets used early and in rates that approximate 1:1 may be helpful in patients with profound hemorrhage (118).
The pelvic retroperitoneum is almost an infinite space and patients can easily exsanguinate into it. The most important initial step in managing pelvic fracture hemorrhage is to reduce the bony pelvis to a normal alignment. This can be accomplished with something as simple as a bed sheet that is wrapped around the patient’s pelvis and tied. In most trauma centers, commercially available binders are now commonly used as the initial hemostatic maneuver.
Angiographic embolization is mainstay of definitive hemostasis. A flush aortic pelvic aortogram often identifies major vascular injuries. Smaller vascular injuries can be identified by selective hypogastric angiography. Once identified, vascular injuries can be occluded with the use of stainless steel coils, gel foam, or a combination of the two. Early embolization is key to prevent coagulopathy and hypothermia. Older patients have a higher likelihood of arterial bleeding with more transfusion requirements (119,120). Some patients require more than one embolization procedure to control hemorrhage (121). CT scanning can be helpful, as it may identify vascular injuries which appear as contrast blush in the pelvis.
In patients who are bleeding too quickly to tolerate the time it takes to mobilize angiographic resources, several other options exist. Extraperitoneal pelvic packing can be helpful. This is a procedure generally performed in the operating room. The pelvic hematoma is entered and evacuated. Small vessel bleeding can be controlled by tightly packing the pelvis with a number of laparotomy pads. This has been shown to be effective but is unlikely to control major hemorrhage (122).
Resuscitative endovascular balloon occlusion of the aorta (REBOA) is a new technique that can be especially useful in patients with pelvic fractures (123,124). A transfemoral catheter is inserted into the common femoral artery via a 12-French sheath. In the case of pelvic fracture hemorrhage, it is inflated just above the aortic bifurcation. This limits pelvic fracture hemorrhage and most patients generally stabilize. This can be left inflated for some time to allow for additional imaging studies, such as CT scanning, as well as time to mobilize angiographic resources, obviating the need for extraperitoneal pelvic packing in some patients. Early work with the REBOA in patients with pelvic fractures has been encouraging (125). At the authors’ center, pelvic fracture hemorrhage is the most common indications for deployment of the REBOA.
Key Points
- Patients presenting after injury should typically have either signs or symptoms to guide diagnosis of their injuries.
- Fractures missed at the time of initial presentation can cause significant morbidity both in the long and short term. Estimates are that fractures are missed up to 10% of the time in multiply injured patients.
- All long bone fractures should be splinted as part of the initial management. Every time the patient is transferred or moved in any way, unsplinted fractures are displaced, producing additional blood loss, inflammation and pain, and risking secondary nerve or vascular injury.
- The physiologic rationale for Damage Control Orthopaedics is based upon the timing and extent of the initial inflammatory response that follows a major injury.
- A difference (Δp) between the systemic diastolic and compartment pressures of 30 mmHg or less is a reliable indicator of impending compartment syndrome and is used by many as an indication for fasciotomy.
- Resuscitative endovascular balloon occlusion of the aorta (REBOA) is a new technique that can be especially useful in patients with pelvic fractures.
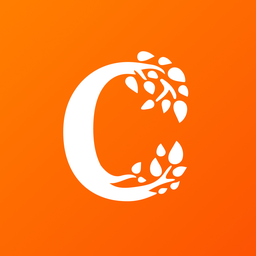
Full access? Get Clinical Tree
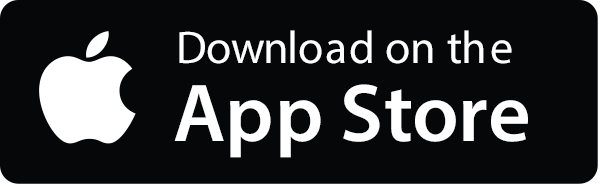
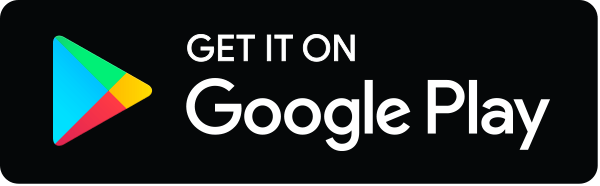