Motion artifact
Poor perfusion due to hypothermia or decreased cardiac output
Nail polish and artificial nails
Skin pigmentation
Cardiac arrhythmias
Electromagnetic interference from surgical cautery
Ambient light
Abnormal hemoglobin variants (methemoglobin)
Poor position or application of the probe
Venous pulsation from tricuspid regurgitation
Intravenous dyes (methylene blue)
21.2.2 End-Tidal Carbon Dioxide Monitoring
End-tidal carbon dioxide (ETCO2) monitoring or capnography displays the patient’s exhaled carbon dioxide concentration continuously during exhalation with the ETCO2 being the peak value before the next breath is initiated. Capnometers estimate ETCO2 through the use of infrared technology such that the concentration of CO2 in the exhaled gas is measured by the absorption of infrared light by CO2. Following its introduction into anesthesia practice, it has become the standard of care to continuously monitor ETCO2 whenever general anesthesia is provided. Additionally, the measurement of ETCO2 via infrared or other technology is standard of care whenever endotracheal intubation occurs. When used continuously in the ICU or operating room setting, the technology provides a continuous estimation of the partial pressure of CO2 (PaCO2) in the blood and serves as a disconnect alarm during mechanical ventilation, monitoring respiratory rate, and providing information regarding pulmonary function via the shape of the capnogram. Abrupt changes in the ETCO2 such as decreases related to increased dead space may alert the clinician to decreased cardiac output or alterations in pulmonary perfusion related to gas or pulmonary embolism. Acute increases in ETCO2 may be the initial sign of malignant hyperthermia or other hypermetabolic states.
The normal capnogram has 3 phases of exhalation and one of inspiration, generally labeled as phases 1–4. Phase 1 is the beginning of exhalation, representing dead space ventilation and therefore having no ETCO2 present. If there is ETCO2 present during phase 1, this is indicative of the rebreathing of exhaled gas, which may be due to inadequate fresh gas flows. Phase 2 is the rapid and steep upslope of the capnogram representing the emptying of alveolar gas with dead space gas. Phase 3 is the plateau phase, which in the normal state should be relatively horizontal. Upsloping of phase 3 of the capnogram indicates obstructive lung disease (asthma, bronchospasm) with differential emptying of alveoli with varying time constants. With the initiation of inspiration, there is an abrupt decrease of the ETCO2 (phase 4) which should return to 0 mmHg.
The ETCO2 generally correlates in a clinically useful fashion with the PaCO2 with the ETCO2 being 2–4 mmHg lower than the PaCO2. This correlation can be used clinically to adjust ventilation techniques both in the operating room and the ICU setting. However, this correlation is dependent on effective matching of ventilation with perfusion, and various technology and patient-related factors may interfere with this accuracy [27–31]. Such issues may be particularly relevant in the practice of pediatric anesthesia where smaller tidal volumes, type of ventilation (continuous versus intermittent flow), and sampling issues can affect the correlation of ET and PaCO2.
Although initially introduced for intraoperative care, capnography has been brought out of the operating room to various clinical arenas. The concern that changes in pulse oximetry may take 60–90 s after the development of apnea in patients receiving supplemental oxygen has led some authorities to recommend the use of continuous ETCO2 monitoring during procedural sedation. While not mandated by all of the current guidelines for procedural sedation, ETCO2 monitoring has been shown to be a beneficial adjunct to monitoring during procedural sedation. While there may be some discrepancy between the ETCO2 value and the PaCO2 especially when monitoring from a nasal cannula, in many cases a direct correlation can be demonstrated [32, 33]. The capnograph provides a continuous and real-time demonstration that air exchange is occurring. If there is upper airway obstruction or central apnea, this is immediately demonstrated as the capnogram extinguishes [34, 35]. Several clinical studies have demonstrated the early identification of respiratory depression using this technology and have clearly indicated its superiority over pulse oximetry in many clinical scenarios in both pediatric and adult patients [36–40]. These data have also been supported by a recent meta-analysis, concluding that episodes of respiratory depression were 17.6 times more likely to be detected by capnography compared with standard monitoring [41]. Owing to the growing evidence, the American Society of Anesthesiologists has amended its standards for basic anesthetic monitoring effective from 2011 to include mandatory ETCO2 monitoring during moderate and deep sedation.
The recent literature and clinical experience clearly demonstrate that capnography is not only effective for confirming ETT placement but may also have an expanded role: the continuous monitoring of tube position in the OR and during transport and monitoring during procedural sedation as a means for providing the immediate identification of apnea or upper airway obstruction. It clearly remains the standard of care for intraoperative monitoring and to document correct endotracheal tube placement wherever endotracheal intubation occurs. Beyond this, it is rapidly becoming the standard of care during procedural sedation especially with the recent revisions of the ASA guidelines. It is also a key monitor in the ICU as a means of rapidly adjusting minute ventilation during mechanical ventilation and for assessing patients with respiratory illnesses. It may provide useful information following endotracheal intubation and during transport as a means of avoiding inadvertent hyperventilation which may be detrimental in patients with traumatic brain injury [42]. More recently, it has been suggested that it can be used to judge the adequacy of resuscitation during cardiac arrest. The depth of chest compression has been shown to correlate with the ETCO2 values [43]. Additionally, higher ETCO2 values during resuscitation have been shown to correlate with a greater chance of return of spontaneous circulation (ROSC). Although initial investigations suggested that an ETCO2 greater than 10 mmHg predicted ROSC, this value has recently been questioned suggesting that the goal ETCO2 during resuscitation should be considered higher, perhaps approaching 25 mmHg [44]. Although ETCO2 was initially applied only in the presence of an ETT, design modifications and the development of new devices allow its application in patients with a native airway during spontaneous ventilation. Given its ability to immediately detect hypercarbia and perhaps respiratory depression, it has been suggested that its use during postoperative period to monitor patients receiving patient-controlled analgesia may lead to the early identification of respiratory depression prior to the development of respiratory arrest thereby improving patient safety [45].
21.2.3 Transcutaneous CO2 Monitoring
Transcutaneous CO2 (TC-CO2) devices can also be used to provide a continuous estimate of PaCO2 in various clinical scenarios. Although introduced for use in the neonatal population where both transcutaneous CO2 and O2 monitoring have been used, these devices have also found their way out of the NICU and into the ICU and OR setting as a means of continuously monitoring ventilation. Their use has been driven by concerns of clinical scenarios that affect the accuracy of ETCO2 or limit their use (high-frequency ventilation techniques). In general, these devices apply heat to the skin at 43–45 °C leading to capillary vasodilatation, increasing the transit time of blood through the capillary, resulting in a close approximation of capillary and arterial PaCO2. The vasodilatation of the capillary bed also allows CO2 to diffuse from the arterial capillary lumen to the membrane of the transcutaneous monitor. Alterations in temperature affect the solubility of CO2 in blood such that an increase in the temperature increases the partial pressure of CO2 in the blood and a higher PaCO2 value or a larger gradient between the actual PaCO2 and the TC-CO2. Additionally, the higher temperature increases the metabolic rate of the tissues, thereby further increasing the PaCO2. These factors are corrected by an internal calibration factor that is used to calculate the TC-CO2 and thereby makes the TC value an appropriate estimate of the PaCO2. Although used less commonly than ETCO2 devices, these devices have seen increased used in various clinical scenarios in both the OR and the ICU setting. These include conventional and high-frequency mechanical ventilation, in spontaneously breathing patients, during the transport of critically ill patients, and in other clinical scenarios including apnea testing during brain death examination and in the assessment of patients with diabetic ketoacidosis (DKA).
In a cohort of pediatric ICU patients with respiratory failure of various etiologies, ranging in age from 1 to 40 months, 100 simultaneously obtained sets of arterial, transcutaneous, and end-tidal CO2 values were analyzed [46]. The end-tidal to arterial CO2 difference was 6.8 ± 5.1 mmHg, while the transcutaneous to arterial CO2 difference was 2.3 ± 1.3 mmHg, p < 0.0001. The absolute difference between the end-tidal and arterial CO2 was ≤4 mmHg in 38 of 100 values, while the absolute difference between the transcutaneous and arterial CO2 value was ≤4 mmHg in 96 of 100 values, p < 0.0001. The same investigators demonstrated the improved accuracy and potential application of TC-CO2 monitoring in an older cohort of patients with respiratory failure who ranged in age from 4 to 16 years of age [47]. A final study evaluated the accuracy of transcutaneous CO2 monitoring following cardiothoracic surgery in infants and children [48]. Given the potential for various physiologic factors including residual shunt and ventilation-perfusion mismatch which may exist following cardiopulmonary bypass (CPB) and surgery for infants with congenital heart disease, the authors speculated that ETCO2 would be inaccurate in this patient population and of limited benefit for continuous monitoring in the pediatric ICU setting. The study population included 33 consecutive patients following surgery for congenital heart disease. Transcutaneous CO2 monitoring was initiated if the initial ABG following CPB demonstrated an arterial to end-tidal gradient of 5 mmHg or more. Although the study validated the utility of TC-CO2 monitoring and its improved accuracy over ETCO2 in this unique patient population, problems with TC-CO2 monitoring were noted in 3 patients who demonstrated cardiovascular instability and were requiring dopamine at 20 μg/kg/min and epinephrine at 0.3–0.5 μg/kg/min. The cutaneous vasoconstriction induced by these vasopressors impacted the accuracy of TC-CO2 monitoring. Other reported ICU and OR applications of TC-CO2 monitoring have included continuous CO2 monitoring during high-frequency ventilation, one-lung ventilation, laparoscopic surgery, apnea testing, and metabolic disturbances where changes in PaCO2 may correlate with acidosis resolution [49–54]. In such settings, ETCO2 may not be feasible due to the ventilation technique or inaccurate due to alterations in pulmonary ventilation-perfusion matching.
As with all noninvasive monitors, attention to detail regarding specific aspects of TC-CO2 monitoring is required to ensure the accuracy of the technique. When compared with ET-CO2 monitoring, TC-CO2 monitoring requires a longer preparation time including a 5-min calibration period prior to placement and then an additional 5–10 min equilibration period after placement on the patient to allow for an equilibration between the transcutaneous and arterial CO2 values. The electrode should be recalibrated and placed at another site every 4 h to avoid burns or blistering of the skin when the device is warmed to 45 °C, while newer devices that attach to the ear lobe and are heated to 43 °C have a lower risk of thermal injury and may be left in place for a longer period of time. While various sites (chest or abdomen) have been used in neonates and infants, we prefer the volar aspect of the forearm in adults especially those with obesity. Accuracy may be affected by technical factors related to the monitor itself including trapped air bubbles, improper placement technique, damaged membranes, and inappropriate calibration techniques. Patient-related issues that affect accuracy include variations in skin thickness; the presence of edema, tissue hypoperfusion, and hypothermia; or the administration of medications that result in cutaneous vasoconstriction. When considering these noninvasive monitors of PaCO2, there are advantages and disadvantages to both (Table 21.2). Given their different clinical utilities, perhaps they should be considered as complimentary rather than competing devices.
Table 21.2
Advantages and disadvantages of transcutaneous and end-tidal devices
Transcutaneous | End-tidal | |
---|---|---|
Advantages | More accurate than end-tidal in many clinical scenarios Easy to use in both intubated and non-intubated patients Accuracy not affected by pulmonary parenchymal disease, shunt, ventilation-perfusion inequalities, type of ventilator, and low tidal volumes Can be used with high-frequency types of mechanical ventilation | Rapid set-up with no calibration Technically easy to use Confirms intratracheal position of ET tube Provides ventilator disconnect alarm. Capnogram provides information regarding pulmonary function |
Disadvantages | More labor intensive than end-tidal devices Requires calibration and placement Must be repositioned every 3–4 h Potential for superficial skin blistering Accuracy affected by decreased perfusion or use of vasoconstricting agents Increasing, but still limited clinical experience outside of the neonatal population | Less accurate than transcutaneous devices in many clinical scenarios Accuracy affected by pulmonary parenchymal disease, shunt, ventilation-perfusion inequalities, type of ventilator, low tidal volumes, and site of sampling Use in non-intubated patients requires specialized sampling device (nasal cannula) |
21.2.4 Acoustic Impedance Monitoring
The latest addition to the respiratory armamentarium is the rainbow acoustic monitoring (RAM, Masimo Corporation, Irvine, California). RAM technology provides continuous, noninvasive monitoring of respiration rate using an innovative adhesive sensor with an integrated acoustic transducer that is externally applied to the patient’s neck. The patented technology, known as Signal Extraction Technology (SET®), separates and processes the respiratory signal to display continuous acoustic respiration rate. Preliminary studies have suggested that this technology is responsive to changes in respiratory rate and is superior to capnography in detecting respiratory pauses. Its use of noninvasive adhesive sensors that are applied externally to the lateral aspect of the patient’s neck may be more easily tolerated than ETCO2 capturing devices. In a study comparing acoustic monitoring with capnography in 33 adults in the post-anesthesia care unit following general anesthesia, the acoustic monitor and capnometer both reliably monitored ventilatory rate, while the acoustic monitor more sensitively identified pauses in ventilation [55]. Although the acoustic monitor was statistically more accurate and more precise than capnography, the clinical differences were modest without proven clinical significance. The authors concluded that acoustic monitoring may provide an effective and convenient means of monitoring ventilatory rate in postsurgical patients. Other studies have demonstrated that respiratory rate monitoring with the acoustic monitor correlates well with that monitored by capnography and that the device is better tolerated than other devices such as a facemask for capnography (Capnomask) [56, 57]. Its preliminary validation and success in these areas have led to its use in the pediatric population for perioperative monitoring and in the adult population outside of the operating room [58–60]. While all of these preliminary studies have suggested that it is better tolerated than capnography by mask or nasal cannula, other advantages over capnography have yet to be determined. Further work is needed to delineate its role in perioperative and postoperative respiratory monitoring.
21.3 Noninvasive Monitoring of Hemodynamic Function
Along with respiratory monitoring (oxygen saturation and ETCO2), the monitoring of cardiovascular parameters and hemodynamic function remains an integral component of standard perioperative monitoring. Although blood pressure monitoring remains a required component of perioperative care, it fails to provide us with even more essential information regarding cardiovascular performance including cardiac output, systemic tissue oxygen delivery, and tissue oxygenation. While these monitors are not considered essential for perioperative care, there remains great interest in the technology that would allow us to noninvasively monitor these parameters.
21.3.1 Cardiac Output Monitoring
The ability to measure cardiac output (CO) at the bedside became a reality in the 1970s, courtesy of the pulmonary artery catheter (PAC) developed by Drs. Swan and Ganz [61]. While the use of a PAC has failed to decrease mortality, there continues to be great interest in CO measurement in the operating room and beyond with the hopes of identifying parameters, which, when altered, will improve outcome [62]. This is evidenced by a Cochrane Database review which concluded that optimizing CO in a goal-directed fashion reduces hospital stay, as well as complications such as respiratory failure, renal impairment, and wound infection [63].
The PAC measures CO by way of the thermodilution technique. Using the thermodilution method, an injectate is administered centrally (right atrium) and a downstream (pulmonary artery) temperature change is measured. The magnitude of the change and its rapidity are directly related to CO. Using a variation of the “Stewart-Hamilton” indicator dilution equation, CO is calculated [62]. Although this method was introduced over 40 years ago, it generally remains accepted as the most accurate method of measuring CO at the bedside. Precision errors as low as 13 % have been reported with the use of PACs in animal models [64]. To eliminate the need for repeated injections which provide only intermittent measures of CO, a PAC was developed with a coil which sits in the right atrium that warms to slightly greater than body temperature, thereby warming the blood and eliminating the need for intermittent injection. Despite their accuracy, PAC use has fallen out of favor with many clinicians given their invasive nature, which may mitigate any clinical advantage their CO measurements bring. In addition, the size of PACs precludes their use in small children, while intracardiac shunts mitigate the accuracy of thermodilution measures of CO, thereby making it even less common among pediatric clinicians.
Transpulmonary thermodilution (TPTD) is another method of CO measurement, which is similar to the PAC. An injectate is administered centrally and a downstream temperature change is measured. However, the technique eliminates some of the invasiveness of the procedure as the distal measurement is measured in the femoral artery rather than the pulmonary artery. This method is likely equivalent in accuracy to the PAC, although it remains an invasive option, requiring the use of specialized central venous and femoral artery catheters [65]. While thermodilution remains the clinical gold standard in CO measurement, it is invasive and often impractical. Given the theoretical benefit of optimizing CO in the perioperative period, newer and less invasive methods of CO measurement are being developed and evaluated.
Doppler techniques offer a noninvasive alternative for the measurement of CO. These monitors are based on the Doppler effect, which states that the change in frequency of a reflected ultrasound signal is proportional to the speed of the reflecting object (blood flow) [66]. These changes in frequency can then be used to calculate velocity and ultimately quantify stroke volume and hence CP. Transthoracic and transesophageal echocardiography use Doppler ultrasound to accurately measure CO; however, they require specialized training and their regular use in the operating room is not feasible [67]. More practical approaches involve esophageal and transcutaneous (suprasternal) Doppler probes. Esophageal Doppler monitors (EDM) are a particularly attractive option in the operative room given its relative ease of placement and lack of interference with most surgical fields. For this reason, it is far more common than suprasternal probes. An EDM simply involves a probe, which is placed in the esophagus and is connected to an analyzer. The probe sends and receives ultrasound signals to the descending aorta, while the analyzer interprets the frequency changes, calculating velocity, which is then used to determine CO. However, up to one third of CO is diverted away from the aorta prior to the point of measurement by an EDM. Additionally, the aortic cross-sectional area is needed to calculate CO based on the measured velocity. These factors are estimated by the analyzer using the patient’s age, weight, and height. Given these limitations, it is not surprising that the absolute values of EDM-measured CO are not interchangeable with the gold standard of thermodilution [68]. However, EDM does consistently show high correlations with thermodilution reference methods. This suggests that EDM may still be useful in the perioperative period by accurately tracking acute changes in CO.
The pulse contour analysis technique involves calculation of cardiac performance based on an arterial waveform tracing. This process necessitates the placement of an arterial catheter and often involves a specialized transducer, which accompanies the signal analyzer. Pulse contour analysis monitors can be categorized as calibrated and non-calibrated varieties. The available calibrated pulse contour monitors use either TPTD or lithium dilution to measure cardiac output [68]. Uncalibrated devices derive CO purely from waveform analysis without input from the other forms of CO measurement. The accuracy of pulse contour analysis remains in question given their significantly smaller body of literature compared with thermodilution and Doppler techniques. With this in mind, the studies suggest that calibrated pulse contour monitors are more accurate when compared to their uncalibrated counterparts. In addition, both types have decreased accuracy during periods of hemodynamic instability [69–73].
Thoracic electrical bioimpedance (TEB) is a completely noninvasive method of monitoring CO, requiring the placement of several electrodes on the external surface of the thorax. This method assumes that resistance to electrical flow is directly related to blood volume within the thorax so that changes in this resistance are used to calculate changes in the intrathoracic blood volume and hence CO. The electrodes are both transmitters and receivers of electrical current, constantly measuring changes in amplitude. The amplitude changes are caused by changes in thoracic fluid volume and ultimately related to descending aortic blood flow. An electrocardiogram measures ventricular ejection time, and the amount of amplitude change during ventricular ejection time is directly proportional to stroke volume. Unfortunately, TEB is prone to errors secondary to pulmonary edema, electrical interference, patient movement, and skin temperature. As such, TEB has shown poor correlation with clinical reference standards [74–76].
Bioreactance is a similar technique, which was developed in an effort to improve on the inaccuracies of TEB. Bioreactance refers to the “phase shift” of voltage, which occurs specifically during pulsatile blood flow. Given the fact that pulsatile flow in the thorax is predominantly taking place in the aorta, bioreactance is able to more accurately measure cardiac output without interference from thoracic fluid (pulmonary edema). Validation studies suggest that bioreactance is far more accurate than TEB when compared with thermodilution [77–79]. However, more research is needed before it can be recommended for routine use.
In conclusion, thermodilution techniques remain the clinical gold standard for cardiac output monitoring. The invasiveness of thermodilution techniques remains their primary drawback; however, no technology to date has proven itself to be superior. Doppler-based techniques, specifically transesophageal Doppler, provide a noninvasive alternative with accuracy that is similar, though not superior, to thermodilution. Calibrated pulse contour analysis is superior to its uncalibrated pulse contour analysis, though both tend to suffer during periods of hemodynamic instability. Bioreactance is completely noninvasive and shows promising accuracy in comparison to thermodilution. It is, however, a relatively newer technology when compared to thermodilution and Doppler counterparts. More research is needed before meaningful conclusions can be made regarding the clinical utility of bioreactance, while bioimpedance monitors have shown poor accuracy and cannot be recommended for clinical use.
21.3.2 Electrocardiogram Monitoring
Continuous monitoring of the electrocardiogram (ECG) remains one of the standard intraoperative monitors during the provision of anesthesia care. Although arrhythmias are uncommon outside of the pediatric cardiac anesthesia arena, bradycardia may occur due to the administration of volatile anesthetic agents (halothane or sevoflurane), hypoxemia, hypothermia, and alterations in intracranial pressure or from the oculocardiac or trigeminocardiac reflex. Although the negative chronotropic effect is less with sevoflurane when compared to halothane, bradycardia may still occur, thereby making continuous ECG monitoring mandatory regardless of the duration of the anesthetic or sedation event [80]. While many of these bradycardic responses are self-limiting, treatment may be required when the heart rate fails to respond to initial therapies such as release of surgical traction or a decrease in the volatile agent concentration. In most clinical scenarios for the pediatric population, a 3-lead ECG is used with the demonstration of lead II to facilitate the identification of P wave morphology and arrhythmia analysis. In adult patients and in specific pediatric patients, a 5-lead ECG is used to facilitate ischemia detection.
Other applications for ECG monitoring include identification of potentially lethal electrolyte disturbances including hyperkalemia, rare instances of prolonged QT syndrome (congenital, acquired, or drug induced), and monitoring for inadvertent systemic injection of local anesthetic agents. Over the years, there has been a dramatic increase in the use of regional anesthesia in infants and children, which has resulted in the increased administration of local anesthetic agents in the age group. Given its prolonged duration of action, the long-acting agent, bupivacaine, is frequently used. As it is relatively cardiotoxic when compared with other agents, a means of identifying inadvertent intravascular or systemic injection is mandatory. To accomplish this, a small amount of epinephrine is generally added to the solution as a test dose [81]. Although this was proposed to result in an increase in heart rate with a high sensitivity, subsequent work demonstrated that when anesthetized with a volatile anesthetic agent, the sensitivity of a heart rate increase was less than expected and not adequate to ensure the safety of the technique. [82–84] By using the T wave, systolic BP, and HR criteria, the positive response rate to an epinephrine test (0.1 mL/kg of a 1:200,000 solution or 0.5 μg/kg) was 100, 95, and 71 %, respectively, during sevoflurane anesthesia and 90, 71, and 71 % during halothane anesthesia [85].
21.3.3 Near-Infrared Spectroscopy
Near-infrared spectroscopy (NIRS) uses infrared light, a technique similar to pulse oximetry, to noninvasively estimate brain tissue oxygenation by measuring the absorption of the light by tissue chromophores (hemoglobin and cytochrome aa3). Pulsatile flow is not required and therefore the device works during CPB and other non-pulsatile states such as cardiopulmonary bypass. Based on the relative absorption of the infrared light at various wavelengths, the specific concentration of the hemoglobin species can be determined using a modification of the Beer-Lambert law [86]. There are two basic types of NIRS monitors: a saturation monitor that measures the difference absorption between oxygenated and unoxygenated hemoglobin and a concentration monitor that measures the concentration of oxygenated and reduced hemoglobin and the relative redox state of cytochrome aa3. The saturation monitor is the one used most commonly in clinical practice and the ones that are currently commercially available for widespread clinical use. To improve the ease of use, instead of providing the specific concentrations of oxygenated and unoxygenated hemoglobin, the NIRS monitors provide a single numeric value known as cerebral oxygenation (rSO2). Cerebral oxygenation monitoring is initiated by placing a self-adhesive probe over the patient’s forehead. Infrared light is directed into the cranium from a light source, and two sensors placed at fixed distances from the light source measure the light after it has passed through extracranial tissue (proximal sensor) or both extracranial and intracranial tissues (distal sensor).
Clinical evidence supports the correlation of the NIRS number (rSO2) with cerebral blood flow (CBF) and oxygenation as changes in rSO2 easily correlate with clinical changes that alter CBF such as blood pressure, PaCO2, and systemic oxygenation. During CPB, rSO2 increases during cooling, with improvements in cardiac output and oxygen delivery. Cerebral oxygenation (rSO2) decreases exponentially and in a predictable and reproducible fashion during deep hypothermic cardiac arrest. Clearly the rSO2 decreases rapidly during periods of hypoxemia, hypocarbia, hypoperfusion, or cardiac arrest. In fact, the rSO2 has been shown to be an earlier and more sensitive indicator of hypoxemia than pulse oximetry [87, 88]. Furthermore, anecdotal experience supports its ability to identify problems related to cannula positioning during CPB. To date, NIRS monitoring has found the greatest use during cardiac surgery in both the adult and the pediatric population. There is accumulating evidence in the adult population which suggests that monitoring rSO2 and acting on changes may alter outcome; however, additional studies are needed to validate these findings in the pediatric population [89–91]. More recent work has demonstrated the use of the NIRS to determine the autoregulatory threshold, demonstrating it to be widely altered in critically ill patients and also suggesting that maintenance of blood pressure within the autoregulatory limits alters outcome [92].
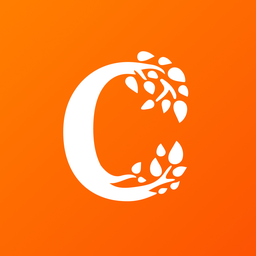
Full access? Get Clinical Tree
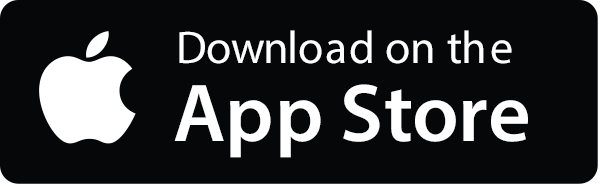
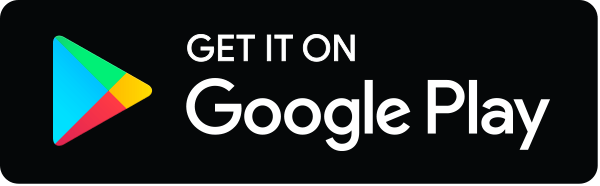