Frank–Starling relationship between ventricular preload and ventricular stroke volume. The first portion of this relationship is called the steep portion and the second portion is called the plateau. If the heart is working on the steep portion (low preload), then an increase in preload (induced by volume expansion) will induce a significant increase in stroke volume (here the heart is said to be preload-dependent). If the heart is working on the plateau (elevated preload), then an increase in preload (induced by volume expansion) will not induce any significant increase in stroke volume (here the heart is said to be preload-independent). The Frank–Starling relationship does not only depend on preload and stroke volume but also depends on ventricular function, and the Frank–Starling curve is flattened when ventricular function is impaired. Consequently, for a given preload value, it is not possible to predict the effects of an increase in preload on stroke volume.
The Frank–Starling relationship does not only depend on preload and stroke volume, but also depends on cardiac function. When cardiac function is impaired, the Frank–Starling relationship is flattened and for the same level of preload the effects of volume expansion on stroke volume are going to be less important, explaining why preload parameters such as CVP or PCWP are not accurate predictors of fluid responsiveness (Figure 16.1). Instead of monitoring a given parameter, functional hemodynamic monitoring assesses the effects of a stress on this given parameter.[19]
Functional hemodynamic parameters
For the assessment of preload dependence, the stress is a “fluid challenge,” and the parameter is stroke volume or one of its surrogates (pulse pressure or plethysmographic waveform amplitude for instance). In mechanically ventilated patients under general anesthesia, the effects of positive-pressure ventilation on preload and stroke volume are used to detect fluid responsiveness. If mechanical ventilation induces important respiratory variations in stroke volume (SVV) or in PPV, it is more likely that the patient is preload-dependent (Figure 16.2).[15]
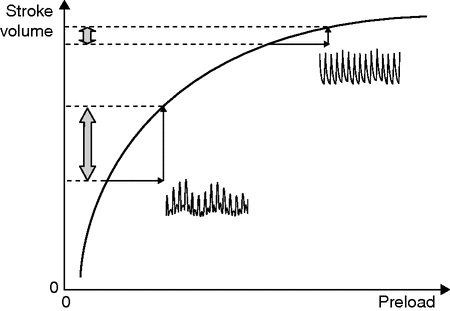
Frank–Starling relationship with associated respiratory variations in the arterial pressure waveform signal. Provided that respiratory variations in arterial pulse pressure are studied in adequate conditions, high respiratory variations reflect that the heart is working on the steep portion of the relationship (indicating a preload dependence), while low respiratory variations reflect that the heart is working on the plateau of the relationship (indicating a preload independence).
These dynamic parameters – SVV, PPV, and passive leg raising (PLR) – have consistently been shown to be superior to static parameters (CVP, PCWP) for the prediction of fluid responsiveness. Today CVP and PCWP, as well as oliguria, hypotension, and tachycardia, should no longer be used for predicting the effects of volume expansion on cardiac output.[18,20]
Theoretically, the main interest of these dynamic parameters is that they can be used as a surrogate for cardiac output monitoring. As a matter of fact, if one considers that knowing whether the patient is preload-dependent (i.e. whether cardiac output can be improved using volume expansion) is more important than knowing the absolute cardiac output value, then monitoring these parameters could replace cardiac output monitoring itself (Figure 16.2).
However, dynamic parameters of fluid responsiveness based on cardiopulmonary interactions have several limitations that need to be clearly stated before they can be adequately used in the clinical setting.
Firstly, these parameters have to be used in mechanically ventilated patients under general anesthesia. Up to now, studies conducted in spontaneously breathing patients have failed to demonstrate that PPV can predict fluid responsiveness in this setting.[21] Moreover, tidal volume has an impact on the predictive value of PPV and a tidal volume of 8 ml/kg of body weight is required.[22] Patients have to be in sinus rhythm, the chest must be closed (open chest as well as open pericardium strongly modifies cardiopulmonary interactions) and the intra-abdominal pressure has to be within the normal ranges.[23]
These dynamic indicators need to be further explored in children and in the setting of left ventricular failure and acute respiratory distress syndrome.
To summarize, the conditions of application of PPV for the purpose of fluid responsiveness are:
patient under general anesthesia and mechanical ventilation;
tidal volume >8 ml/kg of ideal body weight;
sinus rhythm;
no right ventricular failure;
closed chest;
normal intra-abdominal pressure.
Finally, PPV monitoring requires an arterial line and is more likely to be applied in high-risk surgery patients. In moderate-risk surgery patients, the pulse oximeter waveform can be used to assess preload dependence as described below.
Using the pulse oximeter to optimize fluid status
The pulse oximeter waveform is based on a signal proportional to light absorption between an emitter and a receptor, which are usually placed on the fingertip, on the forehead, or on the earlobe. Light absorption increases with the amount of hemoglobin present in the studied tissue.
The amplitude of the pulse oximeter plethysmographic waveform depends particularly on the vessel volume (venous, arterial, and microcirculation volume) and on the transmural pressure applied on the probe.[24] This waveform presents two components: the first component is said to be constant (DC, as direct current) and is due to light absorption by bone, tissue, pigments, non-pulsatile blood (venous), and skin. The second component is said to be pulsatile absorption (AC, as alternating current) and is mainly related to arterial pulse.
Venous blood is responsible for a slight pulsatile absorption on the plethysmographic waveform recorded at the forehead when the pulse oximetry sensor is not pressed by an elastic tensioning headband (i.e. when the transmural pressure is low).[25,26] Without the headband, venous saturation can induce false low-saturation readings. At the finger, the pulsatile component is due primarily to arterialized blood and the venous pulse is less frequently seen. Low-frequency oscillations due to changes in capillary density (sympathetic tone) have also been reported [27] and can alter the waveform quality.
Shamir et al. were the first to describe the respiratory variations in the plethysmographic waveform (SPVpleth) and in its Δdownpleth component (by analogy with the arterial Δdown) in patients presenting with various degrees of hypovolemia.[28] Then, in 2005 our team showed the relationship between the respiratory variations in the ΔPOP and in the PPV in mechanically ventilated patients under general anesthesia in the intensive care unit.[29] In this study, we showed that both indices were strongly related and that ΔPOP was easily measurable at the bedside. Using the PPV formula rather than the variations in the peak of the waveform for quantifying the respiratory variations in the plethysmographic waveform allowed us to get rid of the fact that this waveform has no unit. By dividing the difference in amplitude by the mean of these amplitudes we observe a mathematical simplification for the unit, and then ΔPOP is expressed as a percentage.
Pulse oximeter waveform amplitude was then tested in clinical settings and was shown to be sensitive to venous return in mechanically ventilated patients [30] and to be an accurate predictor of fluid responsiveness in various settings,[13] including in the intensive care unit [31] and in the postoperative period following cardiac surgery.[32] In the operating room, a ΔPOP greater than 13% before volume expansion allowed discrimination between responders and non-responders with 80% sensitivity and 90% specificity.[30]
In the intensive care unit, in a septic hypotensive cohort, Feissel et al. showed that a ΔPOP value of 14% before volume expansion allowed discrimination between responders and non-responders with 94% and 80% specificity, respectively.[31] It is interesting to underline that patients were all under vasoactive drugs in this last study and that it did not seem to impact the ability of this index to predict fluid responsiveness.
We now have numerous evidence showing that ΔPOP has the ability to predict fluid responsiveness in mechanically ventilated patients under general anesthesia despite some limitations related to vasomotor tone.[27] The most recently published study comes from Pizov et al. and shows that respiratory variations in the plethysmographic waveform are early detectors of hypovolemia, and that this parameter increases before arterial pressure decreases and heart rate increases in patients in whom occult bleeding occurs.[33]
Pleth variability index
Most pulse oximeter waveforms displayed by conventional monitor screens are smoothed and filtered because it is impossible to accurately eyeball the respiratory variations in this waveform and to draw any reliable information regarding patients’ fluid status from these screens.[34]
Recently, a new parameter extracted from this waveform allows clinicians to continuously and automatically monitor these respiratory variations. The PVI is continuously displayed as a percentage on Radical 7 Masimo Monitors (Masimo Corp., Irvine, CA). Pleth variability index is an automatic measure of the dynamic change in perfusion index (PI) that occurs during a complete respiratory cycle.
For the measurement of SpO2 via pulse oximetry, red and infrared lights are used. A constant amount of light (DC) from the pulse oximeter is absorbed by skin, other tissues, and non-pulsatile blood, while a variable amount of light is absorbed by the pulsatile arterial inflow (AC). For PI calculation, the infrared pulsatile signal is indexed against the non-pulsatile infrared signal and expressed as a percentage (PI = [AC/DC] × 100) reflecting the amplitude of the pulse oximeter waveform. Then PVI calculation is accomplished by measuring changes in PI over a time interval sufficient to include one or more complete respiratory cycles as PVI = [(PImax − PImin)∕PImax] × 100.
Several studies have now validated the ability of PVI to predict fluid responsiveness in mechanically ventilated patients undergoing general anesthesia.[14,35,36] A PVI higher than 10–15% is suggestive of preload dependence and indicates that volume expansion is more likely to increase cardiac output.
Functional hemodynamic monitoring and clinical outcome
In 2007, the literature regarding the influence of goal-directed therapy based on cardiac output maximization on postoperative outcome was reviewed.[37] The authors concluded that individualized goal-directed therapy in the perioperative period improves gut function and reduces postoperative nausea and vomiting, morbidity, and hospital length of stay.
More recently, a 15-year follow-up study of high-risk surgical patients even found that short-term goal-directed therapy based on cardiac output monitoring in the perioperative period may improve long-term outcomes, in part owing to the ability of such therapy to reduce the number of perioperative complications.[38]
Growing evidence indicates that goal-directed therapy using appropriate fluid monitoring methods, such as PPV and PVI, is effective in optimizing patient outcomes.[15] For example, in a randomized controlled trial published in 2007, monitoring and minimizing PPV by volume loading during high-risk surgery improved postoperative outcome and decreased hospital length of stay.[39] The median duration of postoperative hospital stay was lower in the intervention group than the control group (7 vs. 17 days, p < 0.01), as were the number of postoperative complications per patient (1.4 ± 2.1 vs. 3.9 ± 2.8, p < 0.05), and the median duration of mechanical ventilation (1 vs. 5 days, p < 0.05) and stay in the intensive care unit (3 vs. 9 days, p < 0.01).
Similarly, in a randomized controlled trial in 60 high-risk patients undergoing major abdominal surgery, a goal-directed hemodynamic optimization protocol using the FloTrac/Vigileo device was associated with a shorter median length of stay: 15 days for the goal-directed group vs. 19 days for the control group receiving a standard management protocol (p = 0.006).[10] The goal-directed group also had a reduced incidence of perioperative complications (20%) relative to the control group (50%) (p = 0.03). In another study, high-risk patients undergoing major abdominal surgery whose fluid management was guided by SVV had fewer complications than those receiving routine intraoperative care (p = 0.0066).[40]
PVI may also play a role in optimizing hemodynamic status in patients undergoing major abdominal surgery.[41] In a recently published randomized controlled trial, patients scheduled for major abdominal surgery (n = 82) were randomized into two groups: a PVI group monitored for PVI and a control group receiving standard care. In the PVI group, authors aimed at maintaining PVI under 13% by iterative volume expansion. The primary outcome measure, the perioperative serum lactate level, was significantly lower in the PVI group, and peri- and postoperative volume infused were lower in the PVI group. It was concluded that PVI improved perioperative fluid management in abdominal surgery. Other studies focusing on the ability of non-invasive technologies to be used in goal-directed therapy protocols are now ongoing and should be published in the near future.
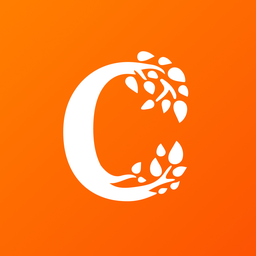
Full access? Get Clinical Tree
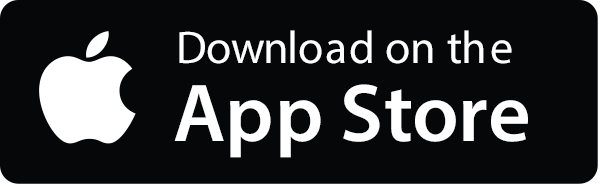
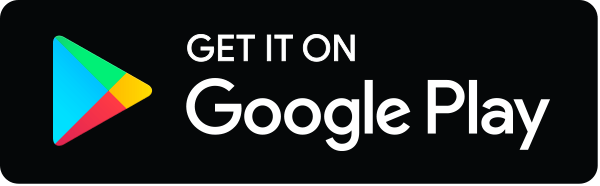