It is generally believed that twitch depression results from block of postsynaptic nicotinic acetylcholine receptors, whereas tetanic or TOF fade results from block of presynaptic nicotinic acetylcholine receptors.10,11 Blockade of the presynaptic nicotinic acetylcholine receptors by neuromuscular blockers prevents acetylcholine from being made available (released from presynaptic nerve terminal) to sustain muscle contraction during high-frequency (tetanic or TOF) stimulation. Because the released acetylcholine does not match the demand, muscle fade is observed in response to stimulation.12
However, there is also strong contrary evidence indicating that fade could be simply a postjunctional (postsynaptic) phenomenon. This latter argument is supported by the fact that the snake toxin, α-bungarotoxin—which binds irreversibly to muscle (postjunctional) nicotinic acetylcholine receptors but does not bind to neuronal (prejunctional) nicotinic acetylcholine receptors—does produce fade.13,14
Depolarizing Neuromuscular Block
In the case of administration of a depolarizing neuromuscular blocking agent, such as succinylcholine, the muscle response that has been “classically” described is quite different. Depolarizing block (also called phase I block) is often preceded by muscle fasciculation. During partial neuromuscular block, depolarizing block is characterized by (a) decrease in twitch tension, (b) no fade during repetitive stimulation (tetanic or TOF), and (c) no posttetanic potentiation (Fig. 12-2).

Pharmacology of Succinylcholine
Structure–Activity Relationships for Succinylcholine
Succinylcholine is a long, thin, flexible molecule composed of two molecules of acetylcholine linked through the acetate methyl groups (Fig. 12-3). Like acetylcholine, succinylcholine stimulates cholinergic receptors at the neuromuscular junction and at nicotinic (ganglionic) and muscarinic autonomic sites, opening the ionic channel in the acetylcholine receptor.

Pharmacokinetics, Pharmacodynamics, and Pharmacogenomics of Succinylcholine
Succinylcholine has an elimination half-life of 47 seconds (95% confidence interval of 24 to 70 seconds).15 The elimination of succinylcholine appears to follow first-order kinetics.15,16 The t½ ke0 and Hill coefficient (after fitting two doses of 1 mg/kg succinylcholine) were 244 seconds (standard deviation 157) and 7.9 (standard deviation 3.3), respectively.15 The dose of succinylcholine causing on average 95% suppression of twitch height (the ED95) is approximately 0.3 mg/kg.17,18
The usual dose of succinylcholine required for tracheal intubation in adults is 1.0 mg/kg. Administration of 1 mg/kg succinylcholine results in complete suppression of response to neuromuscular stimulation in approximately 60 seconds. In patients with genotypically normal butyrylcholinesterase activity, time to recovery to 90% muscle strength following administration of 1 mg/kg succinylcholine ranges from 9 to 13 minutes.19,20 In one study, administration of 0.6 mg/kg of succinylcholine resulted in acceptable intubating conditions at 60 seconds in 95% of patients.21 The reported proportions of patients with acceptable intubating conditions after administration of 1.0 mg/kg succinylcholine varies from 91.8% to 97%.22–24 It also appears that there are no advantages to using succinylcholine doses larger than 1.5 mg/kg in a rapid sequence induction of anesthesia. Paradoxically, succinylcholine doses as large as 2.0 mg/kg guaranteed excellent intubating conditions at 60 seconds in only 86.7% of patients.25 It should be noted that the adequacy of intubating conditions is related not only to the use of a neuromuscular blocker but also to the depth of anesthesia, airway anatomy, and the experience of the laryngoscopist.
The short duration of action of succinylcholine is due to its rapid hydrolysis by butyrylcholinesterase (plasma cholinesterase) to succinylmonocholine and choline, such that only 10% of the administered drug reaches the neuromuscular junction.26 Succinylmonocholine is a much weaker neuromuscular blocking agent than succinylcholine 27 and is metabolized much more slowly to succinic acid and choline.
There is little or no butyrylcholinesterase at the neuromuscular junction. Butyrylcholinesterase influences the onset and duration of action of succinylcholine by controlling the rate at which the drug is hydrolyzed in the plasma before it reaches, and after it leaves, the neuromuscular junction. Recovery from succinylcholine-induced blockade occurs as succinylcholine diffuses away from the neuromuscular junction, down a concentration gradient as the plasma concentration decreases.
Factors Affecting Butyrylcholinesterase Activity
Butyrylcholinesterase is synthesized by the liver and found in the plasma.28,29 The elimination half-time of plasma cholinesterase is 8 to 16 hours, and levels of <75% are necessary for prolongation of succinylcholine effect. Butyrylcholinesterase is responsible for metabolism of succinylcholine, mivacurium, procaine, chloroprocaine, tetracaine, cocaine, and heroin. Neuromuscular block induced by succinylcholine or mivacurium is prolonged when there is a significant reduction in the concentration or activity of butyrylcholinesterase. The activity of the enzyme refers to the number of substrate molecules (µmol) hydrolyzed per unit of time, often expressed in International Units.
Factors that have been described as lowering butyrylcholinesterase activity are advanced liver disease,30 advanced age,31 malnutrition, pregnancy, burns, oral contraceptives, monoamine oxidase inhibitors, echothiophate, cytotoxic drugs, neoplastic disease, anticholinesterase drugs,32,33 metoclopramide,34 and bambuterol.35,36 The β-blocker esmolol inhibits butyrylcholinesterase but causes only a minor prolongation of succinylcholine block.37,38
Neostigmine (and to a lesser degree edrophonium) causes a profound decrease in butyrylcholinesterase activity.39 Even 30 minutes after administration of neostigmine, the butyrylcholinesterase activity remains about 50% of control values. Potent anticholinesterase drugs used in insecticides and occasionally in the treatment of glaucoma and myasthenia gravis, as well as chemotherapeutic drugs (nitrogen mustard and cyclophosphamide), may decrease butyrylcholinesterase activity so that prolonged neuromuscular blockade follows administration of succinylcholine. High estrogen levels, as observed in parturients at term, are associated with up to 40% decreases in butyrylcholinesterase activity. Paradoxically, the duration of action of succinylcholine-induced skeletal muscle paralysis is not prolonged, presumably reflecting an increased volume of distribution of the drug at term.
Genetic Variants of Butyrylcholinesterase
Neuromuscular block induced by succinylcholine or mivacurium can be significantly prolonged if the patient has an abnormal genetic variant of butyrylcholinesterase. Analysis of butyrylcholinesterase involves the determination of both enzyme activity and biochemical phenotypes. Phenotype is determined by the use of specific enzyme inhibitors (such as dibucaine or fluoride) that produce phenotype-specific patterns of dibucaine or fluoride numbers. Molecular genetic analyses can determine the true genotypes. For reviews, see Pantuck 40 and Goodall.41
It is important to recognize that the dibucaine number reflects quality of cholinesterase enzyme (ability to hydrolyze succinylcholine) and not the quantity of the enzyme that is circulating in the plasma. In case of the usual butyrylcholinesterase genotype (E1uE1u), the dibucaine number is 70 or higher, whereas in individuals homozygous for the atypical gene (E1aE1a) (frequency in general population of 1 in 3,500), the dibucaine number is less than 30. In individuals with the heterozygous atypical variant (E1uE1a) (frequency in general population of 1 in 480), the dibucaine number is in the range of 40 to 60.42,43 In individuals with the homozygous atypical genotype (E1aE1a), the neuromuscular block induced by succinylcholine or mivacurium is prolonged to 4 to 8 hours, and in individuals with the heterozygous atypical genotype (E1uE1a), the period of neuromuscular block induced by succinylcholine or mivacurium is about 1.5 to 2 times that seen in individuals with the usual genotype (E1uE1u).44 The longest period of apnea after the administration of succinylcholine was found in patients homozygous for the silent gene (E1sE1s).44 In those patients, TOF stimulation will help in detecting the development of phase II block, a block that may appear after prolonged or repeated administration of succinylcholine and has characteristics similar to nondepolarizing neuromuscular blockers. The decision whether to attempt antagonism of a phase II block has always been controversial and the use edrophonium or neostigmine do not consistently result in adequate antagonism of neuromuscular blockade.45 The alternative is to keep the patient adequately sedated and maintain artificial ventilation until the TOF ratio has recovered to 0.9 or more.
Fluoride-resistant butyrylcholinesterase variants have also been described. In case of the usual butyrylcholinesterase genotype (E1uE1u), the fluoride number is 60, whereas in individuals with the homozygous atypical genotype (E1fE1f), the fluoride number is 36.44 Individuals with homozygous fluoride-resistant genotype exhibit mild to moderate prolongation of succinylcholine-induced paralysis. The heterozygous fluoride-resistant genotype usually produces clinically insignificant prolongation of succinylcholine block, unless accompanied by a second abnormal allele or by a coexisting acquired cause of butyrylcholinesterase deficiency.
Although the dibucaine or fluoride number indicates the genetic makeup of an individual with respect to butyrylcholinesterase, it does not measure the concentration of the enzyme in the plasma nor does it indicate the efficiency of the enzyme in hydrolyzing succinylcholine or mivacurium. Both of these latter factors are determined by measuring butyrylcholinesterase activity—which may be influenced by genotype.
Some rare butyrylcholinesterase variants are associated with increased enzyme activity (two to three times normal).46 Resistance to succinylcholine47 and mivacurium48 as a result of increased butyrylcholinesterase activity has been described.
Side Effects of Succinylcholine
Cardiovascular Effects
Sinus bradycardia, junctional rhythm, and even sinus arrest may follow administration of succinylcholine. These cardiac effects reflect the actions of succinylcholine at cardiac muscarinic cholinergic receptors where the drug mimics the physiologic effects of acetylcholine. Cardiac dysrhythmias are most likely to occur when a second dose of succinylcholine is administered approximately 5 minutes after the first dose. Sinus bradycardia (resulting from stimulation of cardiac muscarinic receptors) is frequently seen in children49 and in adults after a repeated dose of succinylcholine.50 Atropine is effective in treating or preventing bradycardia.
In contrast to actions at cardiac muscarinic cholinergic receptors, the effects of succinylcholine at autonomic nervous system ganglia may produce ganglionic stimulation and associated increases in heart rate and systemic blood pressure. The ganglionic stimulation reflects an effect of succinylcholine on autonomic ganglia that resembles the physiologic effect of acetylcholine at these sites. Ventricular dysrhythmias after succinylcholine administration have been attributed to autonomic stimuli associated with laryngoscopy and tracheal intubation.51
Hyperkalemia
The administration of succinylcholine is associated with approximately 0.5 mEq/dL increase in the plasma potassium concentration in healthy individuals, which is well tolerated and generally does not cause dysrhythmias. Patients with renal failure are no more susceptible to an exaggerated hyperkalemic response to succinylcholine than are patients with normal renal function.52,53
Succinylcholine has been associated with severe hyperkalemia in patients with burn, severe abdominal infections,54 severe metabolic acidosis,55 closed head injury,56 or conditions associated with upregulation of extrajunctional acetylcholine receptors (e.g., hemiplegia or paraplegia, muscular dystrophies, Guillain-Barré syndrome, and burn). For reviews, see Naguib et al.8 and Martyn and Richtsfeld.57 Because of the risk of massive rhabdomyolysis, hyperkalemia, and death in children with undiagnosed muscle disease, succinylcholine is not recommended for use in children except for emergency tracheal intubation.58–60
Myoglobinuria
Damage to skeletal muscles is suggested by the occurrence of myoglobinuria after administration of succinylcholine, especially to pediatric patients.61 It is unlikely that succinylcholine-induced fasciculations could produce muscle damage resulting in myoglobinuria. Most of the patients with rhabdomyolysis and myoglobinuria were subsequently found to have malignant hyperthermia or muscular dystrophy.
Increased Intraocular Pressure
Succinylcholine usually causes an increase in intraocular pressure. The intraocular pressure peaks at 2 to 4 minutes after administration and returns to normal by 6 minutes.62 This increase is likely the result of contraction of tonic myofibrils and/or transient dilatation of choroidal blood vessels. The use of succinylcholine is not widely accepted in open eye injury (when the anterior chamber is open) even though succinylcholine was shown to cause no adverse events in a series of 73 patients with penetrating eye injuries.63 The efficacy of precurarization in attenuating increases in intraocular pressure following succinylcholine administration is controversial.64–66
Increased Intragastric Pressure
Administration of succinylcholine is associated with a variable increase in intragastric and lower esophageal sphincter pressures. The increase in intragastric pressure appears to be related to (a) the intensity of fasciculations of the abdominal skeletal muscles,67 which could be prevented by prior administration of a nondepolarizing neuromuscular blocker; and (b) a direct increase in vagal tone. Administration of succinylcholine does not predispose to regurgitation in patients with an intact lower esophageal sphincter because the increase in intragastric pressure does not exceed the “barrier pressure.”68
Increased Intracranial Pressure
Succinylcholine has the potential to increase intracranial pressure.69 This increase can be attenuated or prevented by pretreatment with a nondepolarizing neuromuscular blocker.70
Myalgias
Postoperative skeletal muscle myalgia, which is particularly prominent in the skeletal muscles of the neck, back, and abdomen, can occur after administration of succinylcholine, especially to young adults undergoing minor surgical procedures that permit early ambulation. Myalgia localized to neck muscles may be perceived as pharyngitis (“sore throat”) by the patient and attributed to tracheal intubation by the anesthesiologist. Muscle pain occurs more frequently in patients undergoing ambulatory surgery, especially in women, than in bedridden patients.71 The incidence of muscle pain following administration of succinylcholine varies from 0.2% to 89%.72 The mechanism of succinylcholine-induced myalgia appears to be complex and is not fully understood. One hypothesis proposes that myalgia is secondary to muscle damage by succinylcholine-induced fasciculations.71 This hypothesis is supported by findings of myoglobinemia and increases in serum creatine kinase level following succinylcholine administration.61,73,74 However, although prior administration of a small dose of a nondepolarizing neuromuscular blocker prevents fasciculations due to succinylcholine, this approach is not always effective in preventing succinylcholine-induced myalgia.72,75 Another hypothesis suggests a possible role for prostaglandins and cyclooxygenases in succinylcholine-induced myalgias.76,77 Pretreatment with a prostaglandin inhibitor (lysine acetylsalicylate or diclofenac) has been shown to be effective in decreasing the incidence of muscle pain after succinylcholine administration.76,78 A meta-analysis showed that myalgia may best be prevented with muscle relaxants, lidocaine, or nonsteroidal antiinflammatory drugs.79 It should be noted, however, that myalgias following outpatient surgery occur even in the absence of succinylcholine.80,81
Masseter Spasm
Succinylcholine is a known trigger agent for malignant hyperthermia. Although an increase in tone of the masseter muscle may be an early indicator of malignant hyperthermia,82 it is not consistently associated with malignant hyperthermia.83–85 It has been suggested that the high incidence of masseter spasm in children given succinylcholine may be due to inadequate succinylcholine dosage.86
Pharmacology of Nondepolarizing Neuromuscular Blockers
Available nondepolarizing neuromuscular blockers can be classified according to chemical class (the steroidal, benzylisoquinolinium, and other blockers) or according to rapidity of onset of action or duration of action (long-acting, intermediate-acting, or short-acting) of equipotent doses (Table 12-1). The pharmacodynamic and pharmacokinetic behaviors of currently used and new nondepolarizing neuromuscular blockers are shown in Tables 12-2 and 12-3, respectively.



Benzylisoquinolinium Compounds
The South American Indians’ arrow poisons, known as curare, served as the basis for the development of the benzylisoquinoline-type relaxants. Tubocurarine, the most important curare alkaloid, was introduced as a neuromuscular blocking drug for use during surgical anesthesia.1,2
Tubocurarine
Tubocurarine is a monoquarternary, long-acting neuromuscular blocker (Fig. 12-4).87 There is no active metabolism of tubocurarine. It is excreted unchanged in the urine, and the liver is probably a secondary route of elimination. Therefore, and because more suitable agents are available, tubocurarine is not indicated for use in patients with either renal88 or hepatic failure. The onset of action of tubocurarine is slow, its duration of action is long, and its recovery is slow (see Table 12-2). The usual intubating dose is 0.5 to 0.6 mg/kg; maintenance doses are 0.1 to 0.2 mg/kg.

Atracurium
Atracurium consists of a racemic mixture of 10 stereoisomers.89,90 These isomers have been separated into three geometrical isomer groups that are designated cis-cis, cis-trans, and trans-trans based on their configuration about the tetrahydroisoquinoline ring system.89,90 The ratio of the cis-cis, cis-trans, and trans-trans isomers is approximately 10:6:1.91
Atracurium has been designed to undergo spontaneous degradation (Fig. 12-5) at physiologic temperature and pH by a mechanism called Hofmann elimination, yielding laudanosine (a tertiary amine) and a monoquaternary acrylate as metabolites.89,92 Furthermore, atracurium can undergo ester hydrolysis. Hofmann elimination is a purely chemical process that results in loss of the positive charges by molecular fragmentation to laudanosine and a monoquaternary acrylate.93,94 Laudanosine depends on the liver for clearance, with approximately 70% excreted in the bile and the remainder in urine.95 Hepatic cirrhosis in humans does not alter clearance of laudanosine, whereas excretion of this metabolite is impaired in patients with biliary obstruction.96 Laudanosine easily crosses the blood–brain barrier, and it has central nervous system stimulating properties. The seizure threshold is not known in humans. In patients in the intensive care unit, blood levels of laudanosine can be as high as 5.0 to 6.0 µg/mL.97 There is no evidence to suggest that prolonged administration of atracurium in the operating room or in the intensive care unit in patients with normal or impaired renal function is likely to result in concentrations of laudanosine capable of producing convulsions. The plasma elimination half-life of laudanosine is similar in patients with normal and impaired renal function—197 ± 38 and 234 ± 81 minutes, respectively.98,99 Interestingly, laudanosine activates α4β2 nicotinic acetylcholine receptors and δ- and κ-opioid receptors. It has been suggested that this activation has neuroprotective effects.100–103

Cisatracurium
Cisatracurium is the 1R cis–1’R cis isomer of atracurium and represents about 15% of the marketed atracurium mixture by weight but more than 50% of the mixture in terms of potency or neuromuscular blocking activity (see Fig. 12-5). Like atracurium, cisatracurium is metabolized by Hofmann elimination to laudanosine and a monoquaternary alcohol metabolite.104–106 There is no ester hydrolysis of the parent molecule.104 Hofmann elimination accounts for 77% of the total clearance of 5 to 6 mL/kg per minute.107 Twenty-three percent of the drug is cleared through organ-dependent means, with renal elimination accounting for 16% of this.106 Because cisatracurium is about four to five times as potent as atracurium, about five times less laudanosine is produced, and accumulation of this metabolite is not thought to be of any consequence in clinical practice. Unlike atracurium, cisatracurium in the clinical dose range does not cause histamine release.108,109 This indicates that the phenomenon of histamine release may be stereospecific.108,110
Mivacurium
Mivacurium is the only currently available short-acting neuromuscular blocker in the European Union, but its use in the United States has been discontinued by the manufacturer (see Table 12-2). Mivacurium consists of a mixture of three stereoisomers. Mivacurium is metabolized by butyrylcholinesterase at about 70% to 88% the rate of succinylcholine to a monoester, a dicarboxylic acid.111,112 Mivacurium may produce histamine release, especially if administered rapidly.113
Steroidal Compounds
The steroid skeleton possesses onium centers at different positions. In the steroidal compounds, it is probably essential that one of two nitrogen atoms in the molecule be quaternized.114 The presence of acetyl ester (acetylcholine-like moiety) is thought to facilitate interaction of steroidal compounds with nicotinic acetylcholine receptors at the postsynaptic muscle membrane.7,115
Pancuronium
Pancuronium is a potent long-acting neuromuscular blocking drug with both vagolytic and butyrylcholinesterase-inhibiting properties (Fig. 12-6).116 About 40% to 60% of pancuronium is cleared by the kidney117 and 11% is excreted in the bile. A small amount (15% to 20%) is metabolized, mainly by deacetylation in the liver. The metabolites (3-OH, 17-OH, and 3,7-di-OH) are considerably less potent as neuromuscular blockers and are excreted in the urine.118 Accumulation of the 3-OH metabolite is responsible for prolongation of the duration of block induced by pancuronium.

Vecuronium
Vecuronium is a monoquarternary neuromuscular blocker with an intermediate duration of action. Vecuronium is simply pancuronium without the quaternizing methyl group in the 2-piperidino substitution (see Fig. 12-6).7,119 The minor molecular difference between vecuronium and pancuronium means that vecuronium is characterized by (a) a slight decrease in potency; (b) virtual loss of the vagolytic properties of pancuronium; (c) molecular instability in solution (this explains in part the shorter duration of action of vecuronium compared with pancuronium); and (d) increased lipid solubility, which results in a greater biliary elimination of vecuronium than pancuronium.7,120
The liver is the principal organ of elimination for vecuronium, and renal excretion accounts for excretion of approximately 30% of the administered dose. Approximately 30% to 40% of vecuronium is cleared in the bile as parent compound.121 The duration of the vecuronium-induced neuromuscular block is therefore dependent primarily on hepatic function and, to a lesser extent, on renal function.122,123 Vecuronium is metabolized in the liver by deacetylation into three possible metabolites: 3-OH, 17-OH, and 3,17-di-OH vecuronium. The 3-OH metabolite has 80% the neuromuscular blocking potency of vecuronium. Therefore, during prolonged administration of vecuronium, this metabolite may contribute to prolonged neuromuscular blockade.124
Vecuronium is prepared as a lyophilized powder because it is less stable in solution. Vecuronium cannot be prepared as a ready-to-use solution with a sufficient shelf life, even as a buffered solution. In pancuronium, the 2-piperidine is quaternized and no longer basic (charged). Thus, it does not participate in catalysis of the 3-acetate hydrolysis.
Rocuronium
Rocuronium (see Fig. 12-6) is an intermediately acting monoquaternary neuromuscular blocker with a fast onset of action than either pancuronium or vecuronium. Rocuronium is about six times less potent than vecuronium.125,126 Rocuronium is primarily eliminated by the liver and excreted in bile. It is taken up into the liver by a carrier-mediated active transport system.127 The putative metabolite, 17-desacetylrocuronium, has not been detected in significant quantities. Approximately 30% of rocuronium is excreted unchanged in the urine.128,129 At room temperature, rocuronium is stable for 60 days, whereas pancuronium is stable for 6 months.
Olefinic (Double-Bonded) Isoquinolinium Diester Compounds
CW002
CW002 (AV002), a benzylisoquinolinium fumarate ester-based compound, is an investigational neuromuscular blocking drug of intermediate duration of action (Fig. 12-7).130 CW002 was designed to undergo cysteine adduction and possibly chemical hydrolysis more slowly than gantacurium. In dogs, administration of 0.08 mg/kg (10× ED95) of CW002 resulted in a duration of action of 71 ± 4 minutes.131 No signs of histamine release were observed in cats in doses up to 0.8 mg/kg (40× ED95).

Potency of Nondepolarizing Neuromuscular Blockers
Drug potency is commonly expressed in terms of the dose-response relationship. The potency of neuromuscular blockers can be expressed as the dose of drug required to produce an effect—for example, 50% or 95% depression of twitch height, commonly expressed as ED50 and ED95, respectively.132 The neuromuscular blocking drugs have different potencies, as illustrated in Table 12-2. The dose-response curve for nondepolarizing neuromuscular blockers is sigmoidal, and different ED values have been derived in a variety of ways. The most commonly used technique is linear regression analysis after log-dose and probit- or logit-data transformation.
Recently, Kopman et al.133 reported that the ED50 value is a very robust parameter and should be employed rather than the ED95 value when comparing the potency of neuromuscular blockers. They noted that the ED50 values calculated by different methods (nonlinear regression analysis, linear regression analysis, or Hill’s equation) were interchangeable, and that the ED95 value was not a precise parameter because the confidence intervals of the ED95 were so wide.133
Factors that Increase the Potency of Nondepolarizing Neuromuscular Blockers
Inhalational anesthetics potentiate the neuromuscular blocking effect of nondepolarizing neuromuscular blockers. This potentiation results mainly in a decrease in the required dosage of neuromuscular blocker and prolongation of both the duration of action of the relaxant and recovery from neuromuscular block.134 The magnitude of this potentiation depends on several factors, including the duration of inhalational anesthesia,135–137 the specific inhalational anesthetic used,138 and the concentration of inhalational agent used.139 The rank order of potentiation is desflurane > sevoflurane > isoflurane > halothane > nitrous oxide–barbiturate–opioid or propofol anesthesia.140–142 The mechanisms proposed for this potentiation include (a) a central effect on α motoneurons and interneuronal synapses,143 (b) inhibition of postsynaptic nicotinic acetylcholine receptors,144 and (c) augmentation of the antagonist’s affinity at the receptor site.145
Some antibiotics can also potentiate neuromuscular blockade. The aminoglycoside antibiotics, the polymyxins, and lincomycin and clindamycin primarily inhibit the prejunctional release of acetylcholine and also depress postjunctional nicotinic acetylcholine receptor sensitivity to acetylcholine.146 The tetracyclines, on the other hand, exhibit postjunctional activity only.146
Hypothermia or magnesium sulfate potentiates the neuromuscular blockade induced by nondepolarizing neuromuscular blockers.147–150 The mechanism(s) underlying this potentiation may be pharmacodynamic, pharmacokinetic, or both.149 High magnesium concentrations inhibit calcium channels at the presynaptic nerve terminals that trigger the release of acetylcholine.8
Most local anesthetics when given in large doses potentiate neuromuscular block; in smaller doses, no clinically significant potentiation occurs.151 Antidysrhythmic drugs, such as quinidine, also potentiate neuromuscular block.152
Factors that Decrease the Potency of Nondepolarizing Neuromuscular Blockers
Resistance to nondepolarizing muscle blockers has been demonstrated in patients receiving chronic anticonvulsant therapy, as evidenced by accelerated recovery from neuromuscular blockade and the need for increased doses to achieve complete neuromuscular blockade. This resistance has been attributed to increased clearance,153 increased binding of the neuromuscular blockers to α1-acid glycoproteins, and/or upregulation of neuromuscular acetylcholine receptors.154
In hyperparathyroidism, hypercalcemia is associated with decreased sensitivity to atracurium and thus a shortened duration of neuromuscular blockade.155 Increasing calcium concentrations also decreased the sensitivity to tubocurarine and pancuronium in a muscle-nerve model.156
Effect of Drug Potency on Speed of Onset
The speed of onset of action is inversely proportional to the potency of nondepolarizing neuromuscular blockers.157,158 Low potency is predictive of rapid onset, and high potency is predictive of slow onset. Except for atracurium,159 molar potency (the ED50 or ED95 expressed in µM/kg) is highly predictive of a drug’s time to onset of effect (at the adductor pollicis muscle).158 Rocuronium has a molar potency (ED95 ≈ 0.54 µM/kg), which is about 13% that of vecuronium and only 9% that of cisatracurium. Thus, rapid onset of rocuronium is to be expected. It should be noted that a drug’s measured molar potency is the end result of many contributing factors: the drug’s intrinsic potency (the CE50, the biophase concentration resulting in 50% twitch depression), the rate of equilibration between plasma and biophase (ke0), the initial rate of plasma clearance, and probably other factors as well.160
The influence of potency on speed of onset could be simply explained by the fact that, for an equipotent dose (e.g., a dose that results in 50% receptor occupancy), a low-potency drug (such as rocuronium) will have a higher number of molecules than a high-potency drug (such as tubocurarine). The higher number of drug molecules will result in a greater diffusion gradient of the low-potency drug from capillary to the neuromuscular junction (faster rate of drug transfer from plasma to biophase) and a greater biophase concentration of low-potency drug resulting in a fast onset.
The concept of “buffered diffusion” must be invoked to explain the slow recovery of long-acting neuromuscular blockers and to understand biophase kinetics.161 Buffered diffusion is the process in which diffusion of a drug (e.g., diffusion of a neuromuscular blocker from the neuromuscular junction) is impeded because it binds to extremely high-density receptors within a restricted space (neuromuscular junction). This process is seen with high-potency but not low-potency drugs. Buffered diffusion causes repetitive binding to and unbinding from receptors, keeping potent drugs such as tubocurarine in the neighborhood of effector sites and potentially lengthening the duration of effect.162,163
Bevan164 also proposed that rapid plasma clearance is associated with a rapid onset of action. The fast onset of succinylcholine is related to its rapid metabolism and plasma clearance.
Adverse Effects of Neuromuscular Blockers
Neuromuscular blocking agents seem to play a prominent role in adverse reactions that occur during anesthesia. The Committee on Safety of Medicines in the United Kingdom reported that 10.8% (218 of 2,014) of adverse drug reactions and 7.3% of deaths (21 of 286) were attributable to neuromuscular blocking drugs.165
Autonomic Effects
Neuromuscular blocking agents interact with nicotinic and muscarinic cholinergic receptors within the sympathetic and parasympathetic nervous systems and at the nicotinic receptors of the neuromuscular junction. Administration of tubocurarine is associated with marked ganglion blockade resulting in hypotension; in susceptible patients, manifestations of histamine release such as flushing, hypotension, reflex tachycardia, and bronchospasm can be seen. Pancuronium has a direct vagolytic effect. Pancuronium can block muscarinic receptors on sympathetic postganglionic nerve terminals,166 resulting in inhibition of a negative-feedback mechanism whereby excessive catecholamine release is modulated or prevented.167 Pancuronium may also stimulate catecholamine release from adrenergic nerve terminals.168
Histamine Release
Histamine release by benzylisoquinolinium compounds such as mivacurium, atracurium, and tubocurarine can cause skin flushing, decreases in blood pressure and systemic vascular resistance, and increases in pulse rate.113,169–172 In contrast, steroidal neuromuscular blocking drugs are not associated with histamine release in typical clinical doses.173–175 The clinical effects of histamine are seen when plasma concentrations increase to 200% to 300% of baseline values, especially if such concentrations are achieved quickly by rapid drug administration. The effect is usually of short duration (1 to 5 minutes), is dose related, and is clinically insignificant in healthy patients. Histamine has positive inotropic and chronotropic effects on the myocardial H2 receptors, and there is some evidence that its chronotropic effect may result in part from the liberation of catecholamines.176 While ganglionic block secondary to the administration of tubocurarine has been demonstrated to occur in various species,177 the peripheral venous and arteriolar dilatation via stimulation of vascular H1 and H2 receptors can result in a significant degree of hypotension as well as carotid sinus–mediated reflex response to histamine-induced peripheral vasodilatation.171 Other substances liberated by mast cell degranulation, such as tryptase or prostaglandins, may also play a role.178 The serosal mast cell, located in the skin and connective tissue and near blood vessels and nerves, is principally involved in the degranulation process.178
For patients who may be compromised hemodynamically, selecting a drug with less or no histamine release (cisatracurium, vecuronium, or rocuronium) may be appropriate. Another strategy for maintaining cardiovascular stability involves slow administration of benzylisoquinolinium neuromuscular blocking drugs (over 60 seconds), or the prophylactic use of the combined histamine H1– and H2-receptor antagonists.
Respiratory Effects
The administration of benzylisoquinolinium neuromuscular blocking drugs (with the exception of cisatracurium) is associated with histamine release, which may result in increased airway resistance and bronchospasm in patients with hyperactive airway disease.
Allergic Reactions
Life-threatening anaphylactic (immune-mediated) or anaphylactoid reactions during anesthesia have been estimated to occur in 1 in 1,000 to 1 in 25,000 administrations and are associated with a mortality rate of about 5%.179,180 In France, the most common causes of anaphylaxis in patients who experienced allergic reactions were reported to be neuromuscular blocking drugs (58.2%), latex (16.7%), and antibiotics (15.1%).181 Anaphylactic reactions are mediated through immune responses involving immunoglobulin E antibodies fixed to mast cells. Anaphylactoid reactions are not immune mediated, and represent exaggerated pharmacologic responses in very sensitive individuals, who represent a very small proportion of the population. Cross-reactivity has been reported between neuromuscular blocking drugs and food, cosmetics, disinfectants, and industrial materials.182
Steroidal compounds (e.g., rocuronium, vecuronium, or pancuronium) result in no significant histamine release.113,175 Nevertheless, in the aforementioned series from France, among cases of anaphylaxis due to neuromuscular blocking drugs, 43.1% were due to rocuronium and 22.6% to succinylcholine.181 There are currently no standards regarding which diagnostic tests (skin prick test, interdermal test, or immunoglobulin E testing) should be performed to identify patients at risk.183
Treatment of anaphylactic reactions includes immediate administration of oxygen (100%) and intravenous epinephrine (10 to 20 µg/kg). Early tracheal intubation with a cuffed endotracheal tube should be considered in patients with rapidly developing angioedema. Fluids (crystalloid and/or colloid solutions) must be administered concurrently. Norepinephrine or a sympathomimetic drug (e.g., phenylephrine) may also be necessary to maintain perfusion pressure until the intravascular fluid volume can be restored. Dysrhythmias should be treated. The use of antihistamines and/or steroids is controversial.
Drugs for Reversal of Neuromuscular Blockade
Acetylcholinesterase at the Neuromuscular Junction
At the neuromuscular junction, acetylcholinesterase is the enzyme responsible for rapid hydrolysis of released acetylcholine.9 Approximately 50% of the released acetylcholine is hydrolyzed during its diffusion across the synaptic cleft, before reaching nicotinic acetylcholine receptors. Acetylcholinesterase has one of the highest catalytic efficiencies known. It can catalyze acetylcholine hydrolysis at a rate of 4,000 molecules of acetylcholine hydrolyzed per active site per second, which is nearly the rate of diffusion.9 Acetylcholinesterase is highly concentrated at the neuromuscular junction but is present in a lower concentration throughout the length of muscle fibers.184
Mechanisms of Action of Acetylcholinesterase Inhibitors
Recovery from muscle relaxation induced by nondepolarizing neuromuscular blockers ultimately depends on elimination of the neuromuscular blocker from the body. Acetylcholinesterase inhibitors (e.g., neostigmine, edrophonium, and less commonly, pyridostigmine) are used clinically to antagonize the residual effects of neuromuscular blockers and to accelerate recovery from nondepolarizing neuromuscular blockade. The acetylcholine that accumulates at the neuromuscular junction after administration of neostigmine competes with the residual molecules of the neuromuscular blocking drug for the available unoccupied nicotinic acetylcholine receptors at the neuromuscular junction. The clinical implication is that neostigmine has a ceiling effect on acetylcholinesterase. Once the inhibition of acetylcholinesterase is complete, administering additional doses of neostigmine will serve no useful purpose because the concentration of acetylcholine that can be produced at the neuromuscular junction is finite. “In practical terms, the maximum depth of block that can be antagonized approximately corresponds to the reappearance of the fourth response to TOF stimulation.”185 Because of their ceiling effect, the anticholinesterases cannot effectively antagonize profound or deep levels of neuromuscular blockade. Indeed, administering more neostigmine at this point may in fact worsen neuromuscular recovery.186 This points to the limitations posed by the use of neostigmine in clinical practice and explains, in part, the high incidence of postoperative residual neuromuscular blockade.187
Antagonism of nondepolarizing neuromuscular blockade by acetylcholinesterase inhibitors depends primarily on five factors: (a) the depth of the blockade when reversal is attempted, (b) the anticholinesterase chosen, (c) the dose administered, (d) the rate of spontaneous clear of the neuromuscular blocker from plasma, and (e) the choice and depth of anesthetic agents administered.188 For a detailed review, see Bevan and colleagues.189,190 For neostigmine, the maximum effective dose is in the 60 to 80 µg/kg range,191,192 and for edrophonium, it is in the 1.0 to 1.5 mg/kg range.193,194 Antagonism of residual neuromuscular blockade induced by the various nondepolarizing neuromuscular blockers is similar (or possibly greater) in children and adults.195 The potencies of different acetylcholinesterase inhibitors in antagonizing the currently available neuromuscular blockers (vecuronium, rocuronium, and cisatracurium) in different clinical scenarios have been reported by several groups of investigators.196–198 Neostigmine is still the anticholinesterase agent most widely used by anesthesiologists worldwide.199
Clinical Pharmacology
Pharmacokinetics of Acetylcholinesterase Inhibitors
The elimination half-life of edrophonium is similar to those of neostigmine and pyridostigmine,200 although that of pyridostigmine is somewhat longer.201,202 Renal excretion accounts for about 50% of the excretion of neostigmine and about 75% of that of pyridostigmine and edrophonium. Renal failure decreases the plasma clearance of neostigmine, pyridostigmine, and edrophonium as much as, if not more than, that of the long-acting neuromuscular blockers.
Side Effects of Acetylcholinesterase Inhibitors
Inhibition of acetylcholinesterase not only increases the concentration of acetylcholine at the neuromuscular junction (nicotinic site) but also at all other synapses that use acetylcholine as a transmitter.
Cardiovascular Side Effects
Because only the nicotinic effects of acetylcholinesterase inhibitors are desired, the muscarinic effects must be blocked by atropine or glycopyrrolate.7 To minimize the muscarinic cardiovascular side effects of acetylcholinesterase inhibitors, an anticholinergic agent should be coadministered with the acetylcholinesterase inhibitor. Atropine (7 to 10 µg/kg) matches the onset of action and pharmacodynamic profile of the rapid-acting edrophonium (0.5 to 1.0 mg/kg),7 and glycopyrrolate (7 to 15 µg/kg) matches the slower acting neostigmine (40 to 70 µg/kg) and pyridostigmine.203,204 In patients with preexisting cardiac disease, glycopyrrolate may be preferable to atropine,205 and the acetylcholinesterase inhibitor and anticholinergic should be administered slowly (e.g., over 2 to 5 minutes). The hemodynamic effects of tracheal extubation are significantly greater than that following the coadministration of neostigmine and glycopyrrolate.
Pulmonary and Alimentary Side Effects
Administration of acetylcholinesterase inhibitors is associated with bronchoconstriction, increased airway resistance, increased salivation, and increased bowel motility (muscarinic effects). Anticholinergics tend to reduce this effect. Findings on whether neostigmine increases the incidence of postoperative nausea and vomiting are discrepant.206,207 Neostigmine has been described as having antiemetic properties208 and as having no effect on the incidence of postoperative nausea and vomiting.207,209
Monitoring of Neuromuscular Function
Monitoring of neuromuscular function after administration of a neuromuscular blocking drug serves at least two purposes in clinical settings. First, it allows the anesthesiologist to administer these agents with appropriate dosing; second, it ensures that the patient recovers adequately from residual effects of the neuromuscular blocker, thus guaranteeing patient safety. In the operating room, the depth of neuromuscular blockade is typically monitored by observing the response to stimulation of any superficial neuromuscular unit. Most commonly, contraction of the adductor pollicis muscle associated with stimulation of the ulnar nerve, either at the wrist or the elbow, is monitored. In certain circumstances, the peroneal nerve or the facial nerve may be monitored.
Clinical bedside criteria for tracheal extubation (such as a 5-second head lift, or ability to generate a peak negative inspiratory force of −25 to −30 cm H2O) are insensitive indicators of the adequacy of neuromuscular recovery.210 Kopman211 noted that patients could sustain a 5-second head lift at a TOF ratio of <0.60. It is recommended that objective monitoring (e.g., digital display of the TOF ratio in real time) be used in the clinical setting. Subjective (visual or tactile) evaluation of the evoked muscular response to TOF stimulation is extremely inaccurate. Subjective evaluation of the evoked muscular response to TOF and tetanic stimulation are notoriously inaccurate as estimates of fade or postoperative residual neuromuscular blockade.212,213 Depth of the blockade during maintenance of and recovery from the blockade should be monitored with repeated TOF stimuli.
Following administration of a nondepolarizing neuromuscular blocking agent, it is essential to ensure adequate return of normal neuromuscular function, to a TOF ratio of ≥0.9. A TOF ratio of <0.9 in unanesthetized volunteers has been associated with difficulty in speaking and swallowing, visual disturbances,211 significant pharyngeal dysfunction resulting in a four- to fivefold increase in the risk of aspiration,214,215 and decrease in hypoxic ventilatory drive.216
Perhaps the most convincing evidence that the use of an objective neuromuscular monitor (combined with a strong educational effort at the departmental level) can decrease the incidence of postoperative residual neuromuscular blockade comes from two studies by Baillard et al.217,218 The first was a prospective study of the incidence of postoperative residual neuromuscular blockade following administration of vecuronium in 568 consecutive patients over a 3-month period in 1995. As was customary in the authors’ department, no anticholinesterase antagonists were used in this series of patients, and peripheral nerve stimulatory devices were rarely used (<2.0%) intraoperatively. Postoperative residual neuromuscular blockade (indicated by an acceleromyographic TOF ratio of <0.70) was present in 42% of patients in the postanesthesia care unit. Of 435 patients who had been extubated in the operating room, the incidence of postoperative residual neuromuscular blockade was 33%.
As a result of these rather alarming findings, Baillard’s department placed acceleromyographic monitors in all operating rooms a short time later. The department instituted a program of education about the use of neuromuscular monitoring and the indications for neostigmine administration. The authors’ findings regarding the incidence of postoperative residual neuromuscular blockade were distributed to department staff. They then conducted repeat 3-month surveys of clinical practice in the years 2000 (n = 130), 2002 (n = 101), and 2004 (n = 218) to determine the success of their educational efforts. In the 9-year interval between the initial and the 2004 survey, the use of intraoperative monitoring of neuromuscular function rose from 2% to 60%, and reversal of residual antagonism increased from 6% to 42% of cases. One other notable change was in the choice of relaxant. In 1995, all patients received vecuronium, but this agent was gradually replaced by atracurium, which was used in 99% of cases in 2004. As a result of these changes in clinical practice, the incidence of postoperative residual neuromuscular blockade (acceleromyographic TOF ratio of <0.90) in Baillard’s department decreased from 62% to <4%.
Although quantitative neuromuscular function monitoring is recommended, many anesthetics are given without such monitoring. Appropriate intraoperative use of a conventional nerve stimulator may decrease (but not eliminate) the incidence of postoperative residual neuromuscular blockade.219 If neostigmine administration is timed at a TOF count of 4, then clinically significant postoperative residual neuromuscular blockade should be a rare event. Quantitative monitors (such as acceleromyography) should be seriously considered in two situations: (a) when no fade on TOF stimulation can be detected manually and the anesthesiologist is deciding not to reverse neuromuscular block or (b) when the anesthesiologist is attempting to reverse deep nondepolarizing block (TOF count ≤3).
Limitations of Acetylcholinesterase Inhibitors
As indicated in the previous sections, postanesthetic morbidity in the form of incomplete reversal and residual postoperative weakness is a frequent occurrence.187,220–224 In 2003, for example, Debaene and colleagues221 reported a 45% incidence of postoperative residual neuromuscular blockade in patients arriving in the postanesthesia care unit. Moreover, a recent survey indicated that most practitioners do not know what constitutes adequate recovery from neuromuscular blockade.225 Although an argument can be made that these problems could be attributed to (a) lack of routine use of peripheral nerve stimulators (and more importantly, the quantitative ones) or (b) the ceiling effect of the reversal agents when administered at a deep level of neuromuscular blockade,226,227 one study found that, despite both the use of nerve stimulators by clinicians with knowledge and expertise and administration of neostigmine, the incidence of critical respiratory events in the postoperative care unit remained a significant 0.8%.222 Clearly, avoidance of critical respiratory events requires changes in clinical care.224
Nonclassic Reversal Drugs
Only a few studies have explored the potential of nonclassic reversal drugs that act independently of acetylcholinesterase inhibition. One such agent, purified human plasma cholinesterase, has been shown to be effective and safe in antagonizing mivacurium-induced neuromuscular blockade.228 Similarly, cysteine has been shown to reverse the neuromuscular blocking effects of gantacurium.229 Sugammadex, a novel selective relaxant-binding agent, is able to reverse both shallow and profound aminosteroid-induced neuromuscular blockade, and has a unique mechanism of action (see the following text) that distinguishes it from cholinesterase inhibitors.
Sugammadex: A Novel Selective Relaxant-binding Agent
Chemistry
Sugammadex is a modified γ-cyclodextrin (Fig. 12-8).230–233 Cyclodextrins are cyclic dextrose units joined through one to four glycosyl bonds that are produced from starch or starch derivates using cyclodextrin glycosyltransferase. The three natural unmodified cyclodextrins consist of 6-, 7-, or 8-cyclic oligosaccharides and are called α-, β-, or γ-cyclodextrin, respectively. Their three-dimensional structures, which resemble a hollow, truncated cone or a doughnut, have a hydrophobic cavity and a hydrophilic exterior because of the presence of polar hydroxyl groups. Hydrophobic interactions trap the drug into the cyclodextrin cavity (the doughnut hole), resulting in formation of a water-soluble guest-host complex.

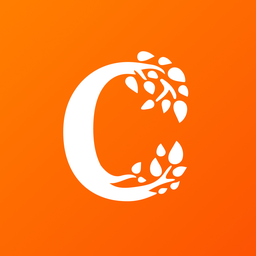
Full access? Get Clinical Tree
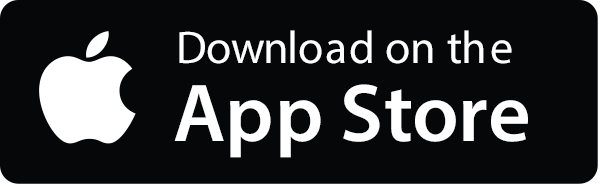
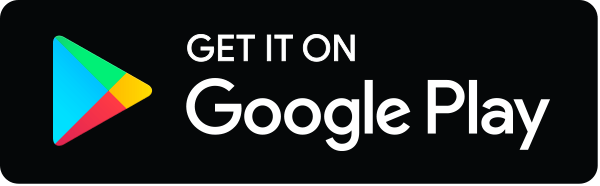