Vishal P. Varshney1,2, Jonathan M. Hagedorn3, & Timothy R. Deer4 1 Department of Anesthesia, Providence Healthcare, Vancouver, British Columbia, Canada 2 Department of Anesthesiology, Pharmacology and Therapeutics, Faculty of Medicine, University of British Columbia, Vancouver, British Columbia, Canada 3 Department of Anesthesiology and Perioperative Medicine, Division of Pain Medicine, Mayo Clinic, Rochester, Minnesota, USA 4 The Spine and Nerve Center of the Virginias, Charleston, West, Virginia, USA Neuromodulation is defined by the International Neuromodulation Society as “the alteration of nerve activity through targeted delivery of a stimulus, such as electrical stimulation or chemical agents, to specific neurological sites in the body” [1]. It involves utilizing implantable devices to modulate abnormal neural pathways with either pharmacologic or electrical stimuli. Neuromodulation therapies can be directed to various anatomical locations along the central and peripheral nervous system. This explains the many forms of neuromodulation therapies that exist today, which include deep brain stimulation, spinal cord stimulation, dorsal root ganglion stimulation, peripheral nerve stimulation and intrathecal drug delivery systems. These therapies have been used to achieve clinical benefit in many disease conditions such as spasticity, epilepsy, peripheral vascular disease, urinary and fecal incontinence and chronic pain [2]. While the applications of neuromodulation are broad, this chapter aims to focus on its application to chronic pain conditions. The history of neuromodulation dates back thousands of years, when ancient Egyptians used the electrical discharge of torpedo fish to treat gout, arthritis and epilepsy [3]. Its applicability with implantable devices began in the early 1960s, first with deep brain stimulation, then with spinal cord stimulation by N. Shealy in 1967 [4]. Over the last few decades, and particularly in the last 5–10 years, the growth in neuromodulation research has been exponential, with significant progress made into our understanding of how neuromodulation works, what anatomical locations are more clinically effective to target and how we can deliver electrical signals, among other areas. Because of this, neuromodulation has emerged as an increasingly valuable and clinically effective chronic pain therapy. Deep brain stimulation (DBS) offers direct stimulation of cortical regions of the brain responsible for pain perception (Figure 23.1). It has been used to manage neuropathic pain resistant to pharmacotherapy for more than 60 years [5]. In patients with centrally mediated pain syndromes, such as post‐stroke pain or neuropathic pain post‐spinal cord injury, thalamic or anterior cingulate cortex (ACC) stimulation has demonstrated limited, but favorable responses [6, 7]. DBS is also indicated in the management of atypical facial pain that has not responded to spinal cord stimulation or peripheral nerve stimulation [6]. Because of limited evidence, this therapy has been used in only end of therapy situations. Figure 23.1 Schematic diagram of a deep brain stimulator system. 1. Implantable pulse generator. 2. Extension cable and burr hole lead. 3. Electrode. Boccard et al. reported a cohort study of 24 patients with refractory neuropathic pain who underwent bilateral ACC DBS [7]. Patient‐reported outcome measures were collected before and after surgery, including the Numerical Rating Scale (NRS), Short‐Form 36 quality of life (SF‐36), McGill Pain Questionnaire (MPQ) and the EuroQol 5‐domain quality of life (EQ‐5D) questionnaire. Six months after surgery, the mean NRS score decreased from 8.00 to 4.27 (p = 0.004). There was a significant improvement in the MPQ (mean, −36%; p = 0.021) and the EQ‐5D score significantly decreased (mean, −21%; p = 0.036). The physical functioning domain of SF‐36 was significantly improved (mean, +54.2%; p=0.01). These findings were sustained at 1 year follow‐up, as NRS score decreased by 43% (P < 0.01), EQ‐5D was significantly reduced (mean, −30.8; P = 0.05) and significant improvements were also observed for different domains of the SF‐36. Patients were followed up to 42 months after implant and efficacy was noted to be sustained, with some patients reporting an NRS score as low as 3. Long‐term stimulation was, however, associated with an increased risk of seizures/epilepsy and this may be related to the pattern of stimulation, which is being investigated by the study authors. The first spinal cord stimulator was inserted in 1967 by N. Shealy, where he performed a thoracic laminectomy to implant a Vitallium electrode measuring 3 by 4 mm, sutured to dura [4]. In the late 1970’s, implantable pulse generators (IPGs) came to market with technology adapted from space satellite power and telemetry systems [8]. This has formed the foundation of what we currently use today, with percutaneous leads developed that allow for superior steering and programming capabilities (8 or 16 contacts) and transcutaneous rechargeable IPGs capable of lasting 5–10 years resulting in reduced morbidity, less frequent IPG replacement and cost savings [9] (Figure 23.2). Figure 23.2 Schematic diagram of a spinal cord stimulator system. 1. Electrode. 2, Implantable pulse generator. The underlying mechanism of how spinal cord stimulation (SCS) works to treat chronic pain has evolved over the last few decades, with Wall and Melzack’s gate control theory thought to be the most accepted proposed mechanism [10]. Gate control theory asserts that stimulation of nonpainful afferent signals “closes the gate” to transmission of painful afferent signals, thereby preventing pain sensations from reaching the brain. Multiple other mechanisms have been elucidated, including: Initially, the only form of SCS available was paresthesia‐based, or tonic, stimulation, where patients would feel paresthesias covering their painful regions. Modification of parameters used to delivery electricity (amplitude, pulse width, frequency) has led to superior forms of SCS, with improved clinical efficacy and less susceptibility to stimulation tolerance and habituation. These forms include non‐linear burst, high‐frequency and differential targeted multiplex stimulation. Each of these forms of stimulation has been validated in robust clinical trials (Table 23.1). Most chronic neuropathic pain conditions have demonstrated response to SCS, such as failed back surgery syndrome, complex regional pain syndrome and chronic peripheral neuropathies [2, 9]. Additional indications include refractory angina, diabetic neuralgia, post‐herpetic neuralgia and visceral or peripheral ischemic pain. Contraindications include active infections, immunosuppression and inability to withhold anticoagulation. Patients with a history of unmanaged psychological and mental health conditions tend to have poorer outcomes and expert mental health assessment is recommended prior to proceeding with trial or device implantation. SCS is typically performed in two phases – a trial phase and a permanent implant phase. Recent evidence has questioned the utility of the trial phase [20]. In the trial period, a percutaneous cylindrical lead or a paddle lead facilitated by laminectomy is inserted under fluoroscopic guidance to the level of the spinal cord corresponding to the patient’s pain area. Trial durations are approximately 5–10 days. A positive trial typically demonstrates at least 50% improvement from baseline pain to proceed to the permanent implant phase. In this phase, either the percutaneous or surgical paddle lead are internalized and attached to an IPG that can be non‐rechargeable or rechargeable. The IPG can be inserted subcutaneously in the posterior flank, buttock or anterior abdominal wall region. Table 23.1 Evidence for novel modes of SCS in recent trials. NPRS: numerical pain rating scale The 2014 Neuromodulation Appropriateness Consensus Committee established that SCS has a low risk of major complications, but minor complications are more common [21]. These typically include electrode fracture or lead migration, which are rectified by either replacing or repositioning the lead. The occurrence rate of lead fracture is estimated to be 5.9–9.1% and lead migration is up to 13.6%. Other complications of SCS include battery/IPG failure (1.7%), biological complications like infection (3.4–10%), epidural hematoma (0.3%) or pain localized to the incision, electrode or IPG site. Although associated with a high upfront cost, SCS has demonstrated cost‐effectiveness overall when compared to conventional medical management (CMM) alone [22–24]. In a variety of disease conditions reviewed, SCS was found to have a significant benefit in incremental cost‐effectiveness ratio (ICER) per quality‐adjusted life years when compared to CMM, which is a generic measure of health benefit that allows the cost‐effectiveness of pain treatments to be compared with other treatments in non‐pain therapy areas as well [22]
Chapter 23
Neuromodulation therapy
Introduction
Deep Brain Stimulation
Spinal Cord Stimulation
History
Review of Mechanisms of Actions
Modes of Stimulation
Indications
Technique
Form of stimulation studied/study name
Study design
Pain presentation studied
Primary outcome
Results
Non‐linear burst
(SUNBURST, 2017) [17]
Multicenter. randomized, controlled, crossover noninferiority trial
Chronic intractable pain of the trunk and/or limbs
Establish non‐ inferiority of pain intensity after three months of burst stimulation compared to three months of tonic stimulation
Burst stimulation is noninferior to tonic stimulation (p < 0.001), and superior to tonic stimulation (p < 0.017)
High frequency
(SENZA, 2015) [18]
Multicenter, randomized, pragmatic, parallel‐arm, noninferiority trial
Chronic, intractable pain of the trunk and/ or limbs
50% or greater back pain reduction with no stimulation‐related neurological deficit
High‐frequency stimulation is superior to tonic stimulation in
reducing back and leg pain (79% vs. 51.3%; p < 0.001)
Differential targeted multiplex (DTM, 2020) [19]
Prospective, multicenter, feasibility study
Chronic intractable back pain with or without leg pain
Change in low back pain relative to a baseline score after a trial period of 3 to 5 days
In DTM group, mean low back NPRS score was 2.4. Difference between mean low back NPRS scores for standard and DTM programming was significant (p < 0.0001)
Complications
Cost‐Effectiveness
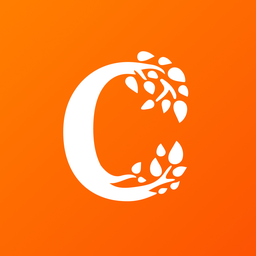
Full access? Get Clinical Tree
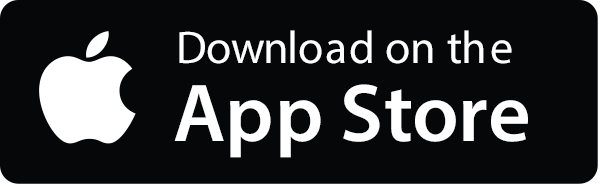
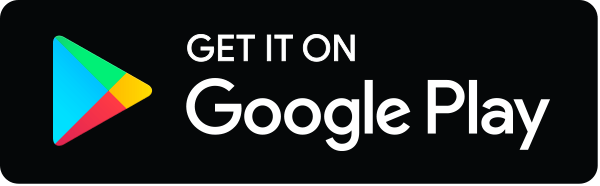