The central nervous system (CNS) coordinates responses to the fluctuating metabolic requirements of the body and modulates behavior, memory, and higher levels of thinking. These functions require diverse cells: astrocytes, neurons, ependymal cells, and vascular endothelial cells. Disruption or death of any one cell type can cause critical changes in the function or viability of another. This cellular interdependence, along with the high metabolic demands of the CNS, makes neurons especially vulnerable to injury from both endogenous neurotoxins and xenobiotics. Endogenous neurotoxins, such as the metals iron, copper, and manganese, are substances that are critical to CNS function but are harmful when their penetration into the CNS is poorly controlled.
The understanding of the normal chemical and molecular functions of the CNS is limited at best. Interestingly, cellular mechanisms are sometimes revealed by investigating xenobiotic-induced neuronal injuries.39,69 For example, the pathophysiology of Parkinson disease, which affects movement and motor tone, was elucidated by the inadvertent exposure of individuals to 1-methyl-4-phenyl-1,2,3,6-tetrahydropyridine (MPTP), a by product of synthesis of a meperidine analog. The mechanisms of axonal transport were elucidated by investigations of the effects of acrylamide exposures in human and animal models.59 The neurodegenerative changes of amyotrophic lateral sclerosis have a promising xenobiotic model in β-methylamino-L-alanine (BMAA), a neurotoxin found in the cyanobacteria associated with cycad plants ingested by the Chamorro people of Guam (Chap. 13).
There are few minimally invasive methods available to investigate xenobiotic-induced CNS injury. Biomarkers are usually nonspecific and not readily available. Xenobiotic concentrations of blood and urine rarely reflect tissue concentrations of the CNS.61 Cerebrospinal fluid (CSF) is useful in excluding CNS injury from infection, hemorrhage, and inflammatory processes, but is, with few exceptions, poorly reflective of the etiology of neuronal injuries.93 Similarly, electroencephalograms and electromyelograms are useful in only a few types of xenobiotic exposures, and neuroimaging, while progressively evolving,55 is a poor substitute for neuroanatomical evaluations that are usually available only on autopsy. Much of the current study to elucidate the mechanisms of CNS injury uses animal models, cultured astrocytes, and other tissue, or postmortem investigations. Less commonly, occupational evaluations, such as the enzyme activity of cholinesterases, are used in pesticide-exposed workers.
This chapter reviews some basic anatomic and physiologic principles of the nervous system and the common mechanisms by which xenobiotics exploit the functional and protective components of the CNS, with a few relevant examples. The multiple factors determining the clinical expression of neurotoxicity are reviewed.
Neurons are the major pathway of cellular communication in the CNS. Having one of the highest metabolic rates in the body, these cells are especially sensitive to changes in the microenvironment and are dependent on astrocytes, choroidal epithelium, and capillary endothelium to confer protection and deliver glucose and other sources of energy.
Although each neuron is capable of receiving information through different neurotransmitters and receptor subtypes at the dendrite, neurons typically produce and release a single type of neurotransmitter at the axonal terminal. This specificity allows for cellular classification of neurons based on the neurotransmitter released, for example, serotonergic, cholinergic, and dopaminergic neurons (Chap. 13). Other substances such as adenosine are produced and released that are less specific to the neuron type.
The anatomic structure of neurons facilitates their function. Dendrites located on the cell body are lined with receptors that bind neurotransmitters and affect cellular changes via several mechanisms. The soma, or cell body, is responsible for coordination and production of multiple proteins required to carry out normal physiological functions. This synthesis occurs at a rate several times greater than that in the liver or kidney. These proteins, organelles, and substrates must then be transported across long distances to the terminal axon. This energy-dependent function is supported by a cytoskeleton composed of neurofilaments, microfilaments, microtubules, and complex transport proteins. Fast anterograde transport of membrane-bound organelles occurs through kinesin, a transport protein, at a rate of 200 to 400 mm/d. Channel proteins, synaptic vesicles, mitochondria, Na+,K+-ATPase, glycolipids, and other substances are transported by kinesin. Slow anterograde transport also occurs, but at a substantially slower rate (0.5–4 mm/d). The retrograde transport protein, dynein, is produced in the soma and delivered to the nerve terminal for the movement of larger vesicles and reusable proteins back to the cell body.
In the CNS, groups of neurons are organized into complex functional pathways, with a single class of neurons regulating different functions and clinical effects depending on the brain region. As an example, dopaminergic neurons regulate cravings, movement, and resting muscle tone, each of which is determined not only by dopaminergic neurons and receptor subtype, but the location in the cortex or basal ganglia (Chap. 13).
Neurons must be able to respond to changes in the local environment and alter the expression of different receptors in response to signaling from neurotrophic factors, variations in metabolic requirements, and xenobiotic interactions. This “neuroplasticity” accounts for the diversity of clinical responses to xenobiotics that induce tolerance such as ethanol (Chap. 14).
Glial cells are composed of astrocytes, oligodendrocytes, Schwann cells, and microglia. These cells serve to support the neurons both structurally and functionally.
Astrocytes comprise between 25% and 50% of the brain volume.1,3,4,12 In addition to the anatomic contribution to the blood–brain barrier (BBB), astrocytes play a critical role in maintaining neuronal function.1,2,96 They contribute to 3 major areas: homeostasis of the extraneuronal environment, provision of energy substrates, and limitation of oxidative stress. In addition, astrocytes contribute to the “plasticity” of cells and receptor expression in the CNS (see later discussion).
In order for cells of all types to function in the CNS, membrane potentials must be adequately maintained. Astrocytes contribute to this by closely regulating the extracellular pH, water, and, like brain capillaries, the extracellular potassium concentration. Metallothioneins, which control the entry of metals necessary for CNS function, are produced by astrocytes.29,96 Astrocytes also release energy substrates such as lactate, citrate, alanine, glutathione, and α-ketoglutarate for utilization by neurons.12
Astrocytes metabolize glutamate, the main excitatory amino acid (EAA) neurotransmitter in the CNS, as well as ammonia. These cells also produce superoxide dismutase and glutathione peroxidase to reduce free radical propagation. Glutathione, the major antioxidant for the brain, is predominantly located in the astrocytes. It is released into the extracellular space or cleaved for neuronal uptake and intracellular reformulation.12
Through the release of complex trophic factors, astrocytes control the expression of endothelial transporters of the BBB and the production of tight junctions in both the blood CSF barrier and BBB. Angiogenesis is similarly astrocyte regulated, as is detection of neuronal injury, immune mediation, and neurotransmitter production. The growth of neurites, the branches of neuronal cell bodies that eventually become dendrites or axons, is similarly modulated by astrocytes.
Oligodendrocytes are a type of glial cell that provide anatomical support, protective insulation, and facilitate rapid neuronal depolarization by the production of myelin. Myelin is the primary constituent of white matter in the CNS. The production of myelin in the peripheral nervous system (PNS) is performed by the Schwann cells. Although oligodendrocytes support several axons, each Schwann cell is anatomically dedicated to a single axon.
Finally, microglial cells modulate immune response, inflammation, and tissue repair from a variety of CNS injuries. Like neurons, microglia are dependent on signaling from astrocytes.
The nervous system has multiple protective mechanisms. Xenobiotics are prevented from accessing the CNS by the blood–brain and blood–CSF barriers. For xenobiotics that enter the CNS, there are multiple cellular specializations to limit oxidant stress as reviewed below.
The BBB confers an anatomic and functional barrier to xenobiotic entry into the CNS. Brain capillaries are surrounded by the foot processes of adjacent astrocytes. The potential spaces between endothelial cells are limited by tight junctions, or zonulae occludentes, which are between 50 and 100 times tighter than those found on peripheral capillaries.1,2,4,53,64 This anatomic barrier prevents movement of substances between cells, also known as the paracellular aqueous pathway, due to osmotic and oncotic forces.1,2,4,53,64
Transendothelial movement of critical substrates and, potentially, xenobiotics occurs through 3 major mechanisms: diffusion, transport proteins, and endocytosis.1,4 These routes allow carefully controlled entry of critical substrates and cofactors while limiting the potential for injury from either endogenous or exogenous neurotoxins.
Lipophilic substances can move directly across the luminal and abluminal endothelial membranes abutting the CNS. Other substances enter the endothelium through bidirectional transport proteins on the luminal surface. Some of these proteins are specific, such as the glucose transporter 1(GLUT1) protein for uptake of glucose. Other less specific large neutral amino acid transporters can move amino acids and xenobiotics such as baclofen, intracellularly. These transporters also line the abluminal surface of the endothelial cell for movement of substrates and xenobiotics into the CNS. The third line of entry for larger proteins is via endocytosis. This is adsorptive, or mediated through specific receptors such as those for insulin or transferrin.1,3,4,53,64,96
Endothelial cells have other protective properties, including intracellular enzymes, to metabolize xenobiotics and efflux proteins to transport certain xenobiotics back into the capillary lumen. These efflux proteins include energy-dependent P-glycoproteins that are ATP-binding cassette transporters and are sometimes referred to as multidrug-resistant (MDR) proteins (Chap. 9). Several hydrophobic xenobiotics are prevented from accumulating in the CNS through these transporters, including vinca alkaloids, digoxin, cyclosporine A, and protease inhibitors. Nonsedating antihistamines and loperamide have limited sedative properties due, in part, to efflux through P-glycoproteins.26,77 Another type of saturable transporter, known as organic acid transport (OAT) protein, facilitates the efflux of hydrophilic xenobiotics such as valproic acid or baclofen. The expression of each of these transporters is upregulated under certain conditions such as intermittent disruptions in the BBB from seizures. This expression upregulation is theorized to account for the resistance of anticonvulsant medications in patients with epilepsy. Comprehensive lists of xenobiotics that are substrates for these transporters are available elsewhere.2,53,54
The ventricles of the brain are lined by the epithelial cells of the choroid plexus. These cells also have tight junctions but not as extensive as those of the BBB. They are, however, rich in glutathione peroxidase and other xenobiotic-metabolizing enzymes in concentrations approximating those of the liver. Similar to brain capillary cells, the choroid plexus contains efflux transporters for organic anions and cations, as well as MDR efflux proteins (P-glycoproteins) to limit entry of xenobiotics into the CSF.1,4,53,96
Neuronal function is strictly dependent on aerobic metabolism. When energy expenditure exceeds production, cellular dysfunction and ultimately cell death, or apoptosis, results. The specific cascade of molecular events relating to this process is termed excitotoxicity.11,74
The initial event is traced to an oxidant stress and excessive stimulation of N-methyl-D-aspartate (NMDA) receptors by glutamate, an EAA neurotransmitter. An influx of intracellular calcium changes membrane potentials across the cellular and mitochondrial membranes. The mitochondria become progressively more inefficient at ATP production and handling the resulting reactive oxygen species. As membrane damage is propagated, calcium further depolarizes the mitochondria, activating a permeability transition pore across the mitochondrial membrane. Gradients are further disrupted, precipitating more injury, energy failure, and ultimately cell death.
Excitotoxicity is considered a common mechanism of cell death due to xenobiotic, ischemic, traumatic, infectious, neoplastic, or neurodegenerative injury. It is the subject of study for many therapeutic interventions in CNS injury.
The clinical expression of neurotoxicity is related to many factors. These include the chemical properties of the xenobiotic, the dose and route of administration, xenobiotic interactions, and underlying patient characteristics including age, gender, and comorbid conditions.
One of the most important determinants of neurotoxicity is the ability of a xenobiotic to penetrate the BBB. Water-soluble molecules larger than 200 to 400 Mr (molecular weight ratio, or mass of a molecule relative to the mass of an atom) are unable to bypass the tight junctions.4 Lipid-soluble xenobiotics (those with a high octanol/water partition coefficient) are more likely to passively penetrate the capillary endothelium, and potentially the BBB, whereas those with a low partition coefficient typically require energy-dependent facilitated transport.64 Xenobiotics that are substrates for capillary endothelial efflux mechanisms will have limited penetration regardless of the coefficient.1,4,53,64
The route of xenobiotic administration is also consequential. Although most xenobiotics gain access to the nervous system through the circulatory system, aerosolized solvents and heavy metals in industrial and occupational exposures gain CNS access through inhalation, traveling via olfactory and circulatory routes. Alternatively, some substances move from the PNS via retrograde axonal transport to the CNS. Naturally occurring proteins such as tetanospasmin, as well as rabies, polio, and herpes virus use this mechanism to access the PNS and CNS.14,17,48 The toxalbumins ricin and abrin as well as bismuth salts also use this mechanism to a limited extent.87,94 This understanding can prove beneficial from a therapeutic perspective. For example, in one small experimental series of patients experiencing severe pain, doxorubicin was injected into the involved peripheral nerves. Therapeutically, a chemical ganglionectomy occurred through retrograde “suicide” transport of doxorubicin, which provided substantial relief for these patients.51
Some xenobiotics are delivered directly into the CSF (intrathecally), the consequences of which are variable (Special Considerations: SC7).
Coadministration of xenobiotics can precipitate neurotoxicity by several mechanisms.46 Extraaxially (outside of the CNS), xenobiotic interactions that result in an increase of the blood concentration of one or both can overwhelm the protective mechanisms of the BBB.21 Similar effects can occur in the PNS, where elevated blood concentrations can enhance clinical effects resulting in peripheral neuropathies.96
Some xenobiotic interactions are synergistic, acting on the same neuroreceptor. Benzodiazepines and ethanol, for example, both stimulate the γ-aminobutyric acid type A (GABAA) receptor. The excessive neuroinhibition results in enhanced sedation and even respiratory depression when these xenobiotics are administered simultaneously.
In some circumstances, xenobiotic interactions result in excessive neurotransmitter availability.10 This neurotransmitter excess is demonstrated in patients with serotonin toxicity, which results from the coadministration of a monoamine oxidase inhibitor and a serotonin reuptake inhibitor or other serotonergics as an example (Chap. 69).
Access to the CNS can be altered by one of the xenobiotics, allowing the other to bypass the BBB. For example, mannitol causes transient opening of the BBB; as a result, therapeutic use of mannitol is under investigation for the delivery of chemotherapeutics that might otherwise be unable to access the nervous system.54 Similarly, some xenobiotics, such as verapamil, cyclosporine, and probenecid, are blockers of capillary endothelium efflux.4,96 These theoretically limit efflux of other substrates of P-glycoprotein or OAT. Loperamide penetration into the CNS is limited by P-glycoprotein. In healthy volunteers the coadministration of quinidine—another P-glycoprotein substrate—with loperamide results in respiratory depression suggestive of increased CNS penetration.77 The clinical utility of employing such efflux blockers is under investigation as was done in a study in which primates received intrathecal methotrexate. The CSF clearance of methotrexate was reduced in animals administered intrathecal probenecid.13,78
Patient-specific variables also affect the ability of a xenobiotic to penetrate the BBB and/or the clinical effects of a given exposure. For example, age of the patient at the time of exposure is critical, especially in the fetus and neonates.80 The structural and enzymatic development of the BBB is incomplete, and synaptogenesis, or formation of intercellular relationships, is especially sensitive to impaired protein synthesis or other excitotoxic events. This is demonstrated classically by maternal exposure to methylmercury. The mother can be minimally affected, but the developing fetus suffers profound neurological and developmental consequences (Chaps. 30 and 95).
In neonates, immature liver function can lead to the accumulation of circulating bilirubin. Because of incomplete formation of the BBB, the bilirubin accesses the CNS and produces a form of encephalopathy known as kernicterus.
Elderly patients are also susceptible to neurotoxins as a result of relatively impaired circulation or age-related changes in mitochondrial function that predispose to excitotoxicity.83 Xenobiotic-induced parkinsonism, or the unmasking of subclinical idiopathic Parkinson disease occurs more readily than in younger patients. Animal models also suggest age-related sensitivity, with one study noting increased manganese toxicity with advanced age.37
Gender is contributory to the expression of xenobiotic induced neurologic injury. In animal models, the presence of estrogen-related and progesterone- related compounds are neuroprotective for some xenobiotic injuries.65,72 In humans, women are more susceptible to some movement disorders such as xenobiotic-induced parkinsonism and tardive dyskinesia, whereas dystonias and bruxism are more prevalent in young men.75 The etiologies of these gender-related differences are incompletely understood.
Conditions affecting the integrity of the BBB affect CNS penetration of xenobiotics and endogenous neurotoxins. For example, glutamate concentrations are normally higher in the circulatory system than the CNS.58 Patients with trauma, ischemia, or lupus vasculitis3 can experience neuropsychiatric disorders as a result of increased penetration of glutamate or sensitivity to additional xenobiotics. Similarly, inflammation associated with meningitis and encephalitis causes openings in the BBB, which is exploited therapeutically. Intravenous penicillin achieves a higher CSF concentration in animals with meningitis than in those without meningitis.79
In some patients, previously undiagnosed diseases become evident on exposure to xenobiotics. This is especially true in patients with peripheral neuropathies. For example, patients being treated with therapeutic doses of vincristine suffered a severe polyneuropathy due to unmasking of a previously undiagnosed Charcot-Marie-Tooth disease.10,23 Similarly, patients with diabetes mellitus, the commonest cause of peripheral neuropathy, or human immunodeficiency virus disease have exacerbation of symptoms in the presence of antiretrovirals.25,73 Patients with myasthenia gravis have exacerbation of weakness with aminoglycoside administration, which affects transmission at the neuromuscular junction (NMJ).95
Chronic exposures to some neuroinhibitory xenobiotics such as ethanol alter neuronal receptor expression and upregulate or increase the amount of receptors for EAAs. In addition to receptor augmentation, neurotransmitter concentrations of the excitatory neurotransmitters glutamate and aspartate are increased, as is homocysteine. These changes induce a tolerance to neuroinhibitory xenobiotics acting on the same receptor, and patients require escalating doses to achieve the same clinical effect. In such patients, cessation of ethanol intake results in a relative deficiency of exogenous inhibitory tone. The patient experiences neuroexcitability and the clinical syndrome of withdrawal18,19 (Chap. 14).
Adequate nutritional status is important for the maintenance of normal neurologic function. The BBB is inadequately maintained in patients with malnutrition. Deficiencies of metal cofactors such as manganese, copper, zinc, and iron affect neurological function. In some cases, the deficiencies enhance absorption of other xenobiotics. For example, iron deficiency enhances lead and manganese absorption in the gastrointestinal tract, which can ultimately overwhelm neuroprotective mechanisms. Vitamins serve as enzymatic cofactors in modulating the production of glutamate, homocysteine, and other amino acids. Specific vitamin deficiencies precipitate neurologic syndromes such as Wernicke encephalopathy in thiamine-depleted patients (Antidotes in Depth: A27 and Chap. 44). The toxicity of xenobiotics is also enhanced. For example, a relative pyridoxine deficiency in patients with acute isoniazid overdose result in seizures as a result of a relative excess of EAAs (Antidotes in Depth: A15 and Chap. 56). Glucose is a critical energy substrate that causes profound neurologic impairment when delivery is inadequate (Antidotes in Depth: A8 and Chap. 47).
Interestingly, certain conditions such as temperature sometimes affect the toxicity of xenobiotics. For example, cooling limits the impedance of acetylcholine neurotransmission caused by botulinum toxin.42
Kidney failure impairs metabolism or elimination of xenobiotics or endogenous substances such as uremic toxins rendering them more available to the CNS. Hyperglycemia in patients with diabetes mellitus increases formation of CNS reactive oxygen species. Similarly, some patients with liver failure have elevations in CNS manganese resulting in Parkinsonism.52,81
Hepatic encephalopathy illustrates the concept of excitotoxicity from endogenous neurotoxins. Hyperammonemia increases oxidative stress and free radical formation in astrocytes. Ammonia potentially decreases critical metabolic enzymes such as catalases, superoxide dismutase, and glutathione peroxidase. Nitric oxide (NO) production is increased as a result of elevations in NO synthetase. Under these conditions, astrocytes upregulate the expression of the peripheral benzodiazepine receptor (PBR) on the outer mitochondrial membrane. The PBR modulates the production of neurosteroids and, in turn, the GABAA receptor. Continued CNS exposure to ammonia and other endogenous solutes propagates this oxidative and nitrosative stress to the mitochondrial membrane, potentially opening the mitochondrial permeability transition pore. Osmotic swelling of the mitochondrial membrane followed by excitotoxicity results in cerebral edema and hepatic encephalopathy.65–67
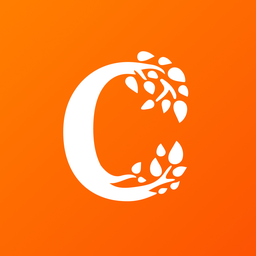
Full access? Get Clinical Tree
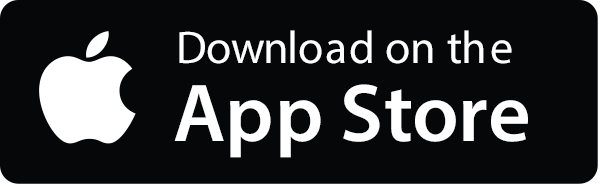
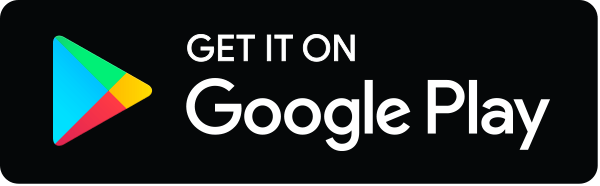