Pearls
- •
Multiple imaging modalities are available for evaluation of the brain, head, neck, and spine of the critically ill child. The most appropriate modality depends on consideration of patient pretest probability for the clinically suspected diagnosis, the modality sensitivity, and the patient’s age and condition.
- •
When ordering radiographic studies, particularly computed tomography scans, the physician should keep the radiation burden in mind, especially for infants. When ordering magnetic resonance imaging for young children, one must keep in mind the risk related to sedation and general anesthesia.
- •
The ever-increasing complexity of imaging modalities and medical problems in the intensive care unit warrants liberal consultation with radiology colleagues to yield an appropriately tailored imaging protocol and a more relevant interpretation.
Imaging modality overview
Multiple imaging modalities are available to investigate the neurologic status of children in the intensive care unit (ICU). To select the most appropriate imaging modality, many factors need to be considered, including the pathologies suspected in a particular case, sensitivity of the imaging modality for the suspected pathologies, complexity of the imaging examination, and the child’s clinical condition. This chapter reviews available imaging modalities and the disease processes that are more commonly evaluated with neuroimaging.
Radiography
The most commonly performed radiographic study in neuroimaging is the radiographic shunt series. The cerebrospinal fluid (CSF) shunt is imaged in the frontal and lateral projections to evaluate the location and course of the proximal catheter, reservoir, valve, and distal catheter in order to detect complications such as disconnection, fracture, improper placement, and migration ( Fig. 61.1 ).

Ultrasound
Standard cranial ultrasound is a mainstay in the neonatal ICU. Ultrasound generates grayscale digital images using sound waves reflecting off tissue interfaces. There is no ionizing radiation and bedside imaging can be performed. Because sound waves cannot readily penetrate the bones of the skull, cranial ultrasound has a restricted window through which sound waves can be used to visualize the brain, primarily through an open anterior fontanelle. Therefore, it is mostly limited to the first few months of life. Ultrasound is sensitive to the detection of germinal matrix hemorrhage, assessment of ventricular size, and identification of moderate to severe white-matter injury. It is less sensitive for pathologies such as mild to moderate parenchymal ischemic changes, subarachnoid and punctate parenchymal hemorrhage, dural venous sinus thrombosis, and cerebral malformations. Extraaxial collections, especially those along the lateral cerebral convexities, can be difficult to visualize on ultrasound performed through a midline fontanelle.
Color Doppler ultrasound uses the shift in frequency associated with reflection of the sound beam off a moving interface (the “Doppler shift” phenomenon) to detect motion in the image field, most commonly from blood in vessels. Those pixels with movement are assigned a color to distinguish them from pixels without movement. The color assigned (most often red and blue) depends on whether movement is away or toward the transducer and does not necessarily indicate arterial or venous flow. Color Doppler allows for some investigation of the cerebral circulation, primarily through the open fontanelle. Transcranial Doppler (TCD) uses the same Doppler shift to produce waveforms that give information about flow velocity and direction. An advantage of TCD is that it can be performed through the thinner portions of the skull, primarily the temporal squamosa, including in older children and adults ( Fig. 61.2 ). TCD has been used in the evaluation of cerebral perfusion in patients with sickle cell disease and vasospasm secondary to subarachnoid hemorrhage (SAH). TCD is generally sensitive to vessel stenosis greater than 50% in the central cerebral circulation, with the highest sensitivity and specificity being in the middle cerebral artery (MCA). , The role of TCD in the ICU is expanding because it enables noninvasive bedside monitoring of cerebral perfusion parameters in various conditions, including in patients on extracorporeal membrane oxygen therapy. However, the utility of TCD in critically ill children requires additional investigation. Moreover, performance of TCD requires specially trained technologists and interpreting physicians.

Computed tomography
Computed tomography (CT) scanning has higher sensitivity compared with ultrasound for many intracranial pathologies, including most neurosurgical emergencies, and is not limited by closing and closed fontanelles. Modern scanners with multiarray detectors image the patient rapidly (decreasing the need for sedation) and acquire volumetric data that may be reformatted in any plane. CT, however, requires transporting the patient from the ICU (although some portable units are available) and uses ionizing radiation (x-rays) to produce digital computer-reconstructed images based on differences in tissue density (which affects x-ray attenuation). The choice of radiation parameters, slice thickness, postprocessing, and image viewing (window width and level) must be tailored to the particular clinical question to optimize the images. For example, CT may be performed at a lower radiation dose when the clinical indication is to evaluate ventricular size. However, this lower-dose CT may not be sensitive to parenchymal abnormalities. Therefore, the ICU team and radiologist need to closely communicate in order to tailor the study appropriately. With advancements in available computational power, newer CT scanners employ complex postprocessing algorithms, such as iterative reconstruction, which enable scanning with lower radiation doses while maintaining image quality. New CT neuroimaging techniques—such as dual-energy CT, in which different energy x-ray sources are simultaneously used—allow improved differentiation of iodine, calcium, and hemorrhage; automated bone removal in CT angiography and CT venography; production of virtual nonenhanced CT images; and metal artifact reduction in the instrumented spine. ,
CT uses differences in density to generate images. Bone and other calcifications have the highest density. Other components in decreasing density are soft tissue (e.g., brain), water (e.g., CSF), fat, and air. On CT, increasing density is represented by an increase in brightness on the images. However, the human visual system is not able to appreciate the entire range of image intensities that are necessary to represent the full range of density values measured by CT. Therefore, the CT images have to be reviewed while adjusting window and level settings to interrogate ranges of density. The difference in density between bone and brain is great, whereas the difference between brain and fat is smaller. The density difference between gray and white matter is much smaller but is sufficient to be appreciated with the appropriate window and level. Generally, as edema develops in nonfatty tissue, there is a decrease in density. Acute blood (approximately between 12 and 72 hours old) is of higher density than brain, and CT is sensitive in the detection of acute (generally less than 7–10 days old) parenchymal, intraventricular, and extraaxial hemorrhage (epidural, subdural, and subarachnoid; Fig. 61.3 ). CT is also useful in the evaluation of ventricular size, extraaxial collections, cerebral edema, mass effect, and cerebral herniation (secondary to space-occupying mass or diffuse cerebral edema).

Iodinated intravenous (IV) contrast can be used with CT to evaluate the integrity of the blood-brain barrier (BBB) and differences in tissue perfusion. With injection of IV contrast, vessels demonstrate a transient increase in density, with a variable rate of contrast leak into the tissues depending on the local pathophysiology. The rate of equilibration of contrast concentration between vessels and parenchyma is much slower in the brain because of the intact BBB. Contrast then is especially useful in the brain, where disruption of the normal BBB can reveal underlying pathology. This increased density from contrast material is also used to image arteries and venous structures with CT angiography (CTA) and CT venography ( Fig. 61.3 C). With the appropriate software, postprocessing of the data can produce three-dimensional (3D) images of the vessels.
Magnetic resonance imaging
Magnetic resonance imaging (MRI) uses a high-field-strength magnet in combination with radiofrequency pulses to produce images based on the nuclear resonance of hydrogen protons in tissue, primarily in water and fat. MRI provides the most sensitive measure of most (but not all) central nervous system (CNS) pathologies. However, imaging requires patient transport to the scanner, increased examination complexity, potential safety concerns related to the strong magnet (typically 1.5 or 3.0 Tesla), and longer imaging time versus CT scan. MRI provides superior tissue characterization because multiple sequences are obtained, each optimized to evaluate certain tissue types or pathologic processes (1–10 minutes required per sequence). MRI is particularly useful in the evaluation of the posterior fossa (brainstem and cerebellum) and spinal cord, where “beam hardening” due to bone results in considerable artifact on CT, although this problem has become less of an issue with modern multidetector CT scanners.
Safety associated with MR scanning is an important issue. MRI-compatible monitoring and life support systems must be used. Patients need to be screened for any internal hardware or implants that would preclude scanning. Electronic devices such as pacemakers are rendered dysfunctional by the changing magnetic field occurring during MRI; hence, patients with such devices generally should not undergo MRI, though MRI may be feasible with some devices in certain situations. Other medical devices, such as cochlear implants, can also limit the feasibility of MR imaging (further details are available at http://www.mrisafety.com ). Many contemporary programmable shunts and vagal nerve stimulators can be scanned but require reprogramming immediately afterward. Injuries and deaths have been reported because of the failure to recognize these dangers.
Most MRI sequences require imaging times in the range of minutes and are susceptible to motion degradation. Though newer sequences have increased tolerance for (minor) motion, for patients who are unable to cooperate, deep sedation or anesthesia is often required. “Fast and feed” approaches taking advantage of postprandial somnolence are often used successfully in young infants, thereby avoiding sedation. It also is important to screen the renal function of patients who might get an MRI because gadolinium-based contrast may need to be used. Gadolinium has been implicated in the development of nephrogenic systemic fibrosis in some patients with compromised renal function, which is not uncommon in the ICU setting. Small amounts of gadolinium can deposit in the body without proven clinical adverse effects; however, ongoing investigation is merited.
MRI exploits nuclear characteristics of hydrogen protons principally in tissue water, referred to as the T1 (longitudinal relaxation) and T2 (spin-spin relaxation) of the tissue. An imaging sequence includes both T1 and T2 components to create an image; however, an individual sequence can be formulated to be predominately one or the other—that is, T1 weighted or T2 weighted (often referred to as simply T1 or T2 sequences). Because most pathobiology results in some disturbance of water, these imaging parameters turn out to be sensitive to many pathologies. On T1-weighted images, water—such as CSF in the ventricles or vitreous in the globes—is dark. This water is bright on T2-weighted images. One point to be aware of is that, for the most part, the signal intensity of any tissue being imaged on MRI is relative (unlike the absolute density measure in CT). Hence, the detection of pathology is dependent on visualizing differences in intensity of one tissue relative to another.
Fluid-attenuated inversion recovery (FLAIR) is a variation of T2-weighted imaging in which the CSF is specifically rendered dark or suppressed to enhance visibility of hyperintense pathology in parenchyma, especially adjacent to normal CSF spaces. Fat is hyperintense on T1-weighted sequences and is also fairly bright on fast T2-weighted sequences, which is possible with most modern-day scanners. In some situations it is advantageous to suppress the brightness of fat, which can be specifically done at the cost of increased scan time. Gradient-recalled echo (GRE) and susceptibility-weighted (SWI) MRI sequences are often used for detection of hemorrhage, although these sequences generally also result in more artifacts. The appearance of acute blood on CT is fairly straightforward, appearing hyperdense initially (because of the extraction of serum), becoming isodense by about 1 week, and appearing hypodense thereafter. The appearance of blood on MRI is more complex. Although it can be detectable for a much longer period on MRI, it can be difficult to appreciate hyperacute hemorrhage (approximately <12 hours old) with MRI, especially SAH. Overall, the detection of calcium and hyperacute blood can be difficult by standard MRI. CT is often better in this regard, though newer sequences, SWI in particular, are sensitive.
IV contrast is also used in MRI, with similar application with CT. In this case the contrast is a gadolinium chelate that shortens the T1 relaxation time of nearby water. The result is that the water near gadolinium is hyperintense on T1-weighted images. This effect can be used to detect BBB disruption, which is seen in various pathologies, including ischemia, inflammation/infection, and status epilepticus. Malignant and some benign brain tumors also demonstrate enhanced capillary permeability with enhancement following contrast. MRI with gadolinium is about an order of magnitude more sensitive than CT using iodinated contrast in detecting BBB disruption. Some areas of the brain lack the BBB, including the choroid plexus, pituitary stalk, the median eminence, and the pineal gland; thus they enhance normally.
Diffusion-weighted imaging (DWI) has assumed an invaluable role, particularly in the intensive care setting. This sequence is now integrated into most brain MRI examinations. It is particularly sensitive to areas where brain parenchyma has sustained acute cytotoxic injury—most commonly, but not limited to, ischemia. DWI is sensitive to the motion of water at the molecular level. Detecting this molecular-level motion requires strong, fast gradients that have become standard on modern MRI scanners to allow sufficiently rapid scanning to effectively “freeze” the gross tissue motion that normally swamps this molecular-level motion. Different cerebral pathologies produce different types of edema (e.g., cytotoxic, vasogenic, and interstitial). Evaluation of the molecular (Brownian) motion of water turns out to be a sensitive measure of some pathologic states, the most significant application currently being cytotoxic edema associated with the detection of ischemia. Cytotoxic edema causes “restriction” of water diffusion with a decrease in the apparent diffusion coefficient (ADC). This is seen as hypointensity on the calculated ADC map image but is more easily appreciated as hyperintensity on the DWI “trace” image. In contrast to cytotoxic edema, vasogenic and interstitial edema result in “increased” water diffusion and therefore an increase in the ADC. Care must be taken in interpreting the DWI trace images because they have some T2 weighting, and “T2 shine-through” can result in a focus of increased signal on DWI trace images that does not represent true restricted diffusion. To confirm true restricted diffusion indicating cytotoxic edema, the calculated ADC map images should be reviewed for corresponding dark signals. Also, the hyperintensity on DWI trace images may pseudonormalize for a period of several days following the ictus because of the development of associated vasogenic edema. Restricted diffusion due to infarct will generally resolve over the course of 1 to 3 weeks; this evolution in diffusion signal allows new infarcts to be distinguished from older lesions. It also should be noted that restricted diffusion is not limited to ischemia and can be seen in other acute cerebral pathologies, primarily myelin vacuolization, as can be seen in acute demyelination, infection, and highly cellular brain tumors.
Advanced magnetic resonance imaging techniques
Newer techniques in MRI, such as MR perfusion, MR spectroscopy (MRS), diffusion tensor imaging, and intracranial vessel wall imaging, are providing new information that is being increasingly used in the management of critically ill patients.
Perfusion MR is used in the evaluation of cerebral perfusion. The most widely available techniques use rapid scanning with a bolus of IV gadolinium contrast to measure first-pass changes in signal intensity. These techniques give a relative measure of perfusion but not quantitative flow. This relative measure can be used to detect diminished cerebral blood flow in one area compared with another. Newer noncontrast MRI techniques, such as arterial spin labeling, generally have lower signal-to-noise ratio (sensitivity) but are improving and potentially quantifiable. There is some early experience with arterial spin labeling in pediatric stroke cases. ,
Conventional MRI is based on the signal intensities derived from the hydrogen bound to oxygen in water and, to a lesser extent, the hydrogen bound to carbon in fat. With the appropriate software, MR can be used as a “probe” for hydrogen bonded to other molecules (termed MR spectroscopy ). The sensitivity is sufficient to detect metabolite molecules in the millimolar range, although at a much lower resolution than that used to detect water for imaging. The nonwater molecules are most commonly reported as ratios of signal peaks that correspond to one of several molecules. In the brain the most common metabolite peaks detected are N-acetyl aspartate, creatine, choline, and—in some physiologic states—lactate. The MRS signal is either obtained from a single voxel (usually several milliliters in volume; voxel is the volume element of the image, which equals pixel × depth) or multiple voxels (as small as 1–2 mL in volume). Multivoxel MRS can be used to make low-resolution images using a technique termed chemical shift imaging for some of the more prevalent metabolite peaks. The utility of MRS is still in evolution, and newer variants of MRS are constantly being evaluated. Detection of lactate to evaluate newborn ischemia, some metabolic diseases, and brain tumors is one of the ongoing applications and areas of research. MRS also has a role in imaging children with mitochondrial disorders and has been applied in creatine deficiency syndromes as two examples of clinical applications.
Magnetic resonance angiography
Fluid motion within tissues can be used by MRI to image flow in vessels and CSF. This imaging can be accomplished with and without contrast, although contrast techniques are generally more sensitive for vascular flow, with some newer contrast angiography sequences also providing temporal/flow information. The MR angiogram can be tailored for artery (MRA) or vein (MRV) visualization, primarily based on flow direction, and is usually most effective if the area of interest can be narrowed—for example, the circle of Willis—although newer techniques have greatly increased the area that can be covered in one scan. The maximum resolution of MRA of slightly under 1 mm is generally less than CTA, where the maximum resolution can be under 0.25 mm. In addition to the source images (the thin angiographic sections obtained during the scan), various reconstructed images can be obtained, including 3D rotational reformations derived from maximum intensity projection reconstructions ( Figs. 61.4 and 61.5 ) and surface renderings, which also can be viewed from multiple projections. Time-resolved MRA techniques (such as time-resolved angiography with interleaved stochastic trajectories), which used the first pass of gadolinium-administered IV, have begun to make inroads in providing temporal information on vascular lesions previously available only with catheter angiograms, though the latter remain the gold standard. Many clinical cerebral arterial questions, however, are now answered with MRA, and MRV of the superficial and deep venous systems has largely replaced diagnostic catheter venograms.

The main indication for MRV is to evaluate for dural venous sinus and cortical vein thrombosis. This may be done either without (i.e., time-of-flight and phase contrast MRV) or with contrast (see Fig. 61.5 ). MRV with contrast is essentially a volumetric 3D gradient recalled echo T1-weighted sequence obtained after the administration of IV contrast. In a recent study by Sari et al., MRV with contrast had a higher sensitivity, specificity, and accuracy in detecting dural venous sinus and cortical vein thrombosis compared with conventional MR sequences and phase contrast MRV.

Catheter angiograms
Although significant progress has been made in cerebral vascular evaluation with both CTA and MRA, catheter angiography remains the gold standard for most CNS arterial imaging when indicated. Catheter angiography also provides temporal/flow data, such as appreciation of early venous drainage with arteriovenous malformations. Angiography is basically a rapid series of radiographs obtained during injection of iodinated contrast directly into the arteries or veins being imaged. Most angiography units produce digital images using digital subtraction (hence the term digital subtraction angiography )—that is, images in which the background “mask” has been subtracted, leaving an image primarily of the contrast-filled vessels. High-end modern-day units use biplane technology that permits acquisition of digital angiographic images from multiple projections using a single-contrast injection. This technology also can be used to derive 3D rotational angiograms, images that can be further postprocessed to obtain volume-rendered and surface-shaded images. Catheter angiography requires transport to and patient support in the angiography suite. Vascular access for arterial studies is usually through the femoral artery and generally has a small but “nonzero” risk of vessel injury, including dissection and embolization. In very young patients, injury to the femoral artery is of greater concern—although any acute risk to the limb is extremely uncommon, relative diminished leg growth in some cases has been reported. Risks of neurologic complication following cerebral angiography are small but do exist. The incidence of permanent defects in diagnostic angiograms using modern techniques is typically reported in the range of 0.5% to 0.7%. , Therapeutic endovascular procedures carry higher risks but generally are in lieu of riskier neurosurgical procedures or, at times, are the only or preferred avenue of treatment. Catheter-based endovascular procedures in children have mostly been limited to the treatment of arteriovenous malformations, fistulas, and aneurysms. However, advancements in adult ischemic stroke intervention using mechanical thrombectomy for revascularization are being investigated in pediatric stroke.
Myelography
Myelography involves radiographs or CT of the spine following opacification of the subarachnoid space by intrathecal injection of iodinated contrast, most commonly injected at the lumbar level. The myelogram has almost completely been replaced by MRI, especially in the pediatric population, because MRI can depict both extrathecal encroachment and intrathecal masses as well as evaluate signal characteristics of the spinal cord itself and any intramedullary mass. High-resolution myelographic type contrast can be achieved using MRI with 3D constructive interference in steady-state and volume T2 sequences to investigate pathologies for which detailed anatomic information is needed, such as with spinal nerve root avulsion and cranial nerve pathologies.
Exceptions for which imaging may revert to CT include the inability to obtain an MRI either because of local field disruption, most commonly from ferromagnetic spinal rods, or because of MRI incompatibility due to safety issues (e.g., a pacemaker). CT provides better results than MRI in the evaluation of the bony spinal column, particularly in patients with trauma, whereas MRI is used in patients with trauma to evaluate soft tissues in the spinal column, primarily the spinal cord.
Nuclear medicine
Nuclear medicine studies involve the administration (injection, ingestion, or inhalation) of a very small amount of radioactively labeled substance (i.e., a radiopharmaceutical) and imaging the localization of the radiopharmaceutical with a gamma camera.
Tc-99m hexamethylpropyleneamine oxime (Tc-99m HMPAO) and Tc-99m ethyl cysteinate dimer are the preferred radiopharmaceuticals for evaluating both cerebral blood flow and perfusion and may be used to support a clinical diagnosis of brain death ( Fig. 61.6 ). Tc99m HMPAO single-photon emission computed tomography (SPECT) may be used to monitor for cerebral perfusion defects in patients with SAH who are at risk for vasospasm. Tc99m HMPAO SPECT with acetazolamide (Diamox), a carbonic anhydrase inhibitor, may be used to evaluate cerebrovascular reserve capacity in patients with chronic cerebral ischemia, such as moyamoya vasculopathy.

A nuclear medicine CSF shunt study may be used in patients with suspected CSF shunt malfunction (such as obstruction of the proximal or distal catheter or valve malfunction). A small amount of radiopharmaceutical is instilled in the shunt reservoir and a combination of dynamic and static images is obtained to follow the distal flow of the radiotracer.
Hypoxic ischemic injury and germinal matrix hemorrhage in the neonate
In the premature infant, ultrasound is the primary modality for detection, grading, and follow-up of germinal matrix hemorrhage (GMH) and its complication, hydrocephalus ( Fig. 61.7 ). GMH is graded as 1 to 4 depending on the presence of intraventricular hemorrhage, ventricular dilation, and hemorrhagic parenchymal venous infarction. Ultrasound may be used to assess for white matter injury, including periventricular leukomalacia (PVL), especially the cystic form. However, MRI will be more sensitive for noncystic PVL. In premature infants, MRI performed at about the third week of life has been reported as predictive of final MRI findings seen at term. Routine screening cranial ultrasound has been recommended for all infants younger than 30 weeks’ gestation between day 7 and day 14, optimally repeated at 36 to 40 weeks’ gestation.

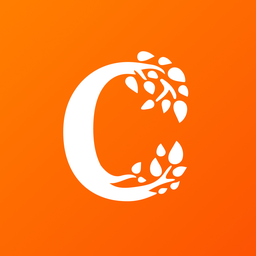
Full access? Get Clinical Tree
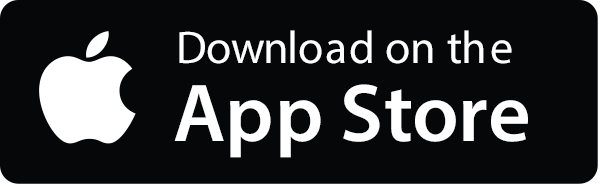
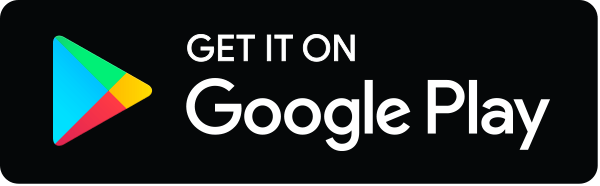
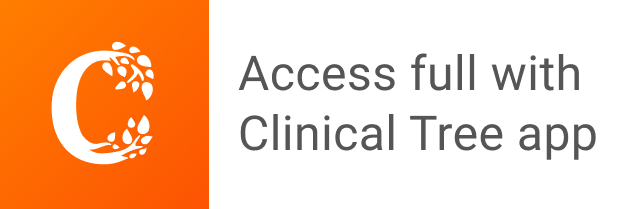