© Springer Science+Business Media New York 2015
Jerrold Lerman (ed.)Neonatal Anesthesia10.1007/978-1-4419-6041-2_99. Neonatal Ventilation
(1)
Paediatric Anaesthesia Unit, Geneva Children’s Hospital, Geneva, Switzerland
Keywords
Neonatal ventilationHypo- and hyperventilationVentilation-induced lung injuryBronchopulmonary dysplasiaHypocapniaHyperventilationPediatric anesthesiaAdequately ventilating the lungs is crucial in neonates since both hypo- and hyperventilation may confer respiratory and systemic consequences, in addition to contributing to the increased morbidity and mortality in pediatric anesthesia [1, 2]. Recently, enormous efforts have been expended to improve ventilation strategies in neonates using a “protective” and “open-lung” strategy in order to maintain optimal functional residual capacity (FRC) and to prevent ventilation-induced lung injury and bronchopulmonary dysplasia. An increased awareness of the potential harm of hyperventilating the lungs of neonates with large tidal volumes (Vt) that can lead to alveolar overdistension due to excessive shear forces and the liberation of proinflammatory cytokines, which constitute the main features of the so-called ventilation-induced lung injury, have caused a reappraisal of such a practice [3, 4]. Moreover, the resultant hypocapnia from hyperventilation may induce cerebral vasoconstriction and promote the development of cystic periventricular leukomalacia [5]. On the other hand, suboptimal Vt may result in inefficient gas exchange, hypercapnia, and hypoxemia and an increased risk of intraventricular hemorrhage (IVH) [6]. To address these problems, several interesting ventilation modes are now available that can optimize tidal volume and meet the ventilation requirements of the neonate. Despite the introduction of several new ventilation strategies, no single ventilation strategy has proven to be superior over the others in terms of neonatal pulmonary and neurologic outcomes. For every ventilation strategy, real-time pulmonary monitoring should be used in order to compensate for sudden changes in the compliance and resistance of the respiratory system. This chapter reviews the specificities of the respiratory characteristics of neonatal pulmonary function, describes the different ventilation modes available for neonates, and highlights the importance of using a protective and open-lung ventilation strategy.
Neonatal Respiratory System
Three specific physiological characteristics distinguish the pulmonary system in the neonate. First, the chest wall, which is comprised of the respiratory muscles and skeletal structures, is very compliant because the ribs are cartilaginous. The horizontal ribs are responsible for the cylinder shape of the infant’s thorax, which contrasts with the elliptical-shaped thorax and bucket-handled orientation of the ribs in older children. Moreover, the effectiveness of the intercostal muscles is limited, supporting the chest wall and minimizing its distortion rather than contributing to the increases in tidal volume as occurs in childhood. Thus, the principal muscle responsible for ventilation in the neonate is the diaphragm. However, the diaphragm in the neonate is disadvantaged as it has a smaller muscle mass, has a reduced content of high-endurance (fast twitch 1) muscle fibers, and inserts more horizontally into the chest wall in infants than in older children [7]. This mechanical disadvantage of the chest wall and diaphragm is exaggerated during respiratory distress when oxygen demand is increased. In this case, contraction of the diaphragm causes the lower rib cage to cave inward, thereby reducing the lung volume and offsetting the effect of the diaphragmatic contraction. Together, these chest wall weaknesses limit the neonate’s ability to generate an adequate alveolar ventilation since most of the force generated is lost on chest wall deformation and fades quickly due to muscle fatigue [8].
The second important pulmonary feature that distinguishes the neonate is the so-called stiff lung. This results from the increased static elastic recoil pressure. The elastic recoil of the lung depends on the amount of elastic fibers within the lung and the surface tension generated at the air–liquid interface within the alveoli [9]. Surfactant reduces the surface tension within alveoli while maintaining patent terminal airways, independent of the alveolar diameter [10]. Moreover, the increased elastin/collagen ratio in neonates increases the forces that tend to collapse alveoli. These forces are counterbalanced in part by the outward opposing forces that prevent the lungs from collapsing. However, because the chest wall is very compliant, the net effect of these two opposing forces reduces the resting lung volume at end expiration. This corresponds to the functional residual capacity (FRC) and represents the resting volume of the respiratory system. Consequently, both the full-term neonate and the preterm infant have a relatively small FRC, even in the presence of efficient surfactant. However, when the amount of surfactant is reduced or respiratory distress syndrome is present, there is a further tendency for the terminal airways to collapse with subsequent atelectasis, loss of lung volume, ventilation-perfusion mismatch, and hypoxemia. This phenomenon is further exaggerated in preterm infants in whom collateral airflow cannot occur at the bronchoalveolar level because of the immaturity of the terminal airways and disturbed alveolarization [11, 12].
Thirdly, the neonatal respiratory system is challenged by the greater resistances of the upper airways and conducting airways of the lung compared with those in older children. The resistance in the upper airways can be substantive in neonates as the airflow is turbulent, and the resistance to airflow during turbulent flow increases with the fifth power of the decrease in radius. Hence, a 50 % reduction in the radius of the airway increases airflow resistance by 32-fold. In contrast, airflow in the smaller distal conducting airways of the lung (beyond the fourth or fifth bronchial division) is laminar. In these distal airways, resistance follows Poiseuille’s equation for laminar flow and is inversely proportional to the fourth power of the radius of the airway). Thus, the radius of the airway is the most important variable in airway resistance, which explains the significant increase in the work of breathing in neonates when inflammatory disorders and thickened mucous membrane from respiratory infections or secretions are present. The gradual increase in airway resistance that occurs from the reductions in the airway radius from successive airway generations is offset by the greater cross-sectional area of the airways. In addition, studies have consistently demonstrated a reduction in airway resistance with increasing height [13, 14], whereas in neonates, airway resistance increases from airway closure and the loss in lung volume.
When considering the total respiratory system resistance, it is important to appreciate that the tracheal tube and the resistance from the forces generated by the viscoelastic component of the lung account for more than two-thirds of the total resistance. In neonates, the use of tracheal tubes with small diameters increases the respiratory resistance, and the relatively high elastic recoil of the lung may further exacerbate the resistive component of the respiratory system [13]. Thus, when ventilating the lungs of neonates, the pressure delivered to the lung must overcome both the frictional forces of the airway resistance as well as the tissue elastic recoil and the chest compliance. Many clinical conditions often observed in neonates alter ventilation and jeopardize our ability to successfully ventilate the lungs under anesthesia. Accordingly, positive-pressure ventilation of the lungs in the presence of decreased compliance such as after surfactant deficiency or congenital diaphragmatic hernia may become a serious challenge [15]. In addition, other congenital or acquired diseases (tracheomalacia, bronchial infection, and inflammation) may lead to increased resistance to air flow and require specific ventilation modes in order to obviate very high airway pressures that may contribute to ventilation-induced lung injury.
Ventilation Modes
Our expanding insight into neonatal respiratory pathophysiology combined with advances in ventilator technology has led to the introduction of a range of sophisticated ventilation modes, the impact of which has not yet been fully appreciated primarily because of the lack of randomized trials. Although a number of ventilation modes are used routinely in the neonatal intensive care unit (NICU), they have confusing terminology and are not available in anesthesia workstations. The available ventilation modes can be distinguished according to whether they are volumetric (e.g., flow generator), barometric (the pressure generator), or dual mode, a combination of both pressure and flow generators. Newer anesthetic ventilators allow the child to trigger the onset of the ventilator cycle, which further expands the number of ventilator strategies available [16].
Pressure-Controlled Ventilation
Pressure-controlled ventilation (PCV) is the most popular mode for use in neonates in clinical practice [16]. The basic and most frequently applied mode in NICU is the time-cycled, pressure-limited ventilation (also called intermittent positive-pressure ventilation: IPPV), a mode that is present on most anesthesia ventilators as PCV. The decelerating flow and the limited and constant inspiratory pressure that characterize this mode explain the reduced peak inspiratory pressure with attenuated tracheal and alveolar pressures. In addition, this mode compensates for a potential leak around an uncuffed tracheal tube, still routinely used in neonates. The combination of decelerating flow and constant airway pressure over time facilitates the equilibration of tracheal and alveolar pressures, which improves the distribution of ventilation and, thereby, improves gas exchange [17]. However, in PCV mode, three factors should be considered when selecting a tidal volume: (1) the pressure gradient between the maximum inspiratory set pressure (peak inspiratory pressure: PIP) and the end-expiratory positive pressure (PEEP); (2) the inspiratory time (T i), which may depend and/or determine the ratio between the inspiratory and expiratory time as well as the respiration rate; and (3) the time constant, which depends on both the compliance and resistance of the total respiratory system and determines the time to equilibrate the pressures within the airways and alveoli. Therefore, when applying the PCV mode, in addition to limiting the peak inspiratory pressure (PIP) and the level of the PEEP, the settings for this mode require that the inspiratory and expiratory times (T e) be adjusted in order to determine the respiration rate, irrespective of the child’s own breathing, but usually at a level close to that observed physiologically. Whether T i is large or small does not affect the incidence of chronic lung disease in neonates, although a brief T i may decrease the risk of an air leak and decrease mortality [18]. Thus, T i is usually adjusted to 0.35–0.5 s, with a greater T e, the latter determining the respiration rate. However, during anesthesia, apart from increasing the level of PEEP, T i can be increased if a greater mean airway pressure is required without causing asynchronous breathing between the child and the ventilator [19] that may occur in the absence of heavy sedation. Conversely, a smaller T i during weaning is more advantageous in neonates. To overcome this limitation, synchronized ventilation with the neonate allowed to trigger the onset of inspiration is currently the most popular ventilation mode in the NICU. Among the different triggering techniques that have been developed, flow triggering via a flow sensor interposed between the tracheal tube and the ventilator’s connection is the most sensitive trigger [20] and is therefore widely used in clinical practice. Synchronized intermittent mandatory ventilation (SIMV), pressure control mode, and assist-control (AC) modes have been introduced in the intensive care settings in order to assist the child’s respiratory effort by securing a fixed respiration rate synchronized with the child’s breathing (SIMV) or by assisting each breath on the basis of positive-pressure ventilation, but with a “control” of a minimum number of ventilator cycles (AC). If the child breathes rapidly, the initial T i causes the ventilator to assist every triggered breath, which may decrease T e and lead to air trapping from an inadequate expiratory time. This risk is also observed when using pressure-support ventilation (PSV), a mode that is present on most new anesthesia ventilators. In the PSV mode, both initiation and termination of ventilator assistance are controlled by the child’s respiratory effort and changes in the airflow. Depending on the type of ventilator, inflation ceases when the inspiratory flow level decreases to between 10 % and 25 % of the maximum inspiratory flow [21]. This mode is very interesting in anesthesia and has been used in older children to reduce the work of breathing (WOB) associated with spontaneous respiration and to counteract the large resistance due to small diameter tracheal tubes. Because of the greater risk of patient-ventilator asynchrony in critically ill neonates with tachypnea, PSV may increase oxygen consumption and lead to ineffective respiratory efforts. A novel technique known as proportional assist ventilation (PAV) has been developed to prevent such phenomena from developing. This technique offers the advantage of further reducing the WOB. In this mode, the rate of lung inflation and thus the inspiratory pressure are controlled by the child and are proportional to the child’s inspiratory effort [22]. Since the pressure applied depends on the inspiratory flow generated by the child, this mode assumes that neither hypopnea nor a leak around the tracheal tube is present [19]. However, this mode is not available on anesthesia machines. More interesting is the neurally adjusted ventilator assist (NAVA), which relies on the child’s respiratory control and diaphragmatic activity [23, 16]. The inspiratory effort is detected via bipolar electrodes mounted on a nasogastric feeding tube, positioned at the level of the diaphragm. The level of ventilatory support is then proportional to the inspiratory effort. Although this mode is unaffected by the presence of a leak around the tracheal tube, its utility is still not defined in neonates, especially in premature infants with immature control of the respiration. In a retrospective study of NAVA compared with SIMV-PC in premature infants <1,500 g, NAVA resulted in better blood gas regulation, oxygen requirements, and reduced peak inspiratory pressure [24]. A recent prospective crossover study demonstrated that NAVA is associated with improved patient-ventilator synchrony and reduced peak airway pressure in comparison with PSV [25]. Moreover, a similar study demonstrated a reduced peak inspiratory pressure, inspired oxygen requirements, and respiratory rate to achieve a reduced carbon dioxide tension and better compliance after NAVA compared with PCV [26].
Volume-Controlled Ventilation
The major disadvantage of pressure-limited ventilation lies in the variable tidal volume (Vt). This results from changes in lung compliance and resistance that occur constantly in the neonate, particularly during general anesthesia. Thus, some anesthesiologists prefer volume-controlled ventilation (VCV), a mode that is based on the traditional delivery of a fixed preset Vt at a preestablished rate. This mode does not take into account the peak airway pressure that is needed to deliver the desired Vt and the magnitude of the tracheal pressures that may be encountered during ventilation, especially in the presence of reduced lung compliance or the increased airway resistance. Moreover, this mode of ventilation requires manual adjustment of the Vt to compensate for the compression of the gas within the ventilator circuit and gas leak around the uncuffed tracheal tube. These increased pressures may be attenuated by either setting the pressure pop-off valve or by adjusting the T i in order to limit excessive pressures in neonates with chronic lung disease.
Volume-Targeted Ventilation
The increasing awareness of the usefulness of direct control of the PIP and the benefit of ventilation with a small constant Vt has led to the development of dual ventilation modes that guarantee Vt with a limited pressure [27]. Different ventilators with novel modes have been developed to permit the choice of both a target Vt and an adjustable pressure limit, the latter permitting the ventilator to autoregulate the inspiratory pressure (within the maximum limit set) or the T i to guarantee the target Vt. These modalities are routinely used in NICU and are slowly finding their way into the operating room. These modes are known as volume-targeted ventilation modes that guarantee Vt by measuring the exhaled Vt and adjusting the peak inflation pressure to deliver the target Vt [28]. However, there is no evidence that volume-targeted ventilation offers any advantages over pressure-limited ventilation in terms of outcomes such as the risk of chronic lung diseases [29]. Nonetheless, volume-targeted ventilation has been associated with a significantly smaller duration of ventilation, a decreased rate of pneumothorax, and a decreased incidence of severe (grade 3 or 4) IVH when compared with pressure-limited ventilation [29, 30]. These modes are now available on some new anesthetic ventilators, but there is a dearth of studies on their clinical usefulness in anesthesia, despite their advantages.
Among these interesting novel ventilator modes, the volume guarantee (VG) ventilation mode is in fact a pressure-limited, volume-targeted time, or flow-cycled ventilator that merits further attention. The working pressure in this ventilation mode is adjusted to minimize the difference between the exhaled Vt (measured by the ventilator) and the desired Vt (set by the clinician) on a breath-by-breath basis. This mode can also be used in combination with other standard modes available for use in neonates including SIMV, AC, or PSV. When setting the parameters in this mode, not only should the desired Vt be taken into account but so should the T i in order to determine the duration of insufflation based on the time-constant concept from pressure-controlled ventilation. In addition, the maximum peak inspiratory pressure should exceed the working pressure, but at a limit that protects the lungs from any risk of barotrauma should the lung compliance suddenly decrease. These ventilator characteristics are particularly interesting for the weaning period since the inspiratory pressure is adjusted in real time [27]. Other anesthesia machines have integrated the pressure-regulated volume control (PRVC) mode, where flow rate varies with the inspiratory pressure to deliver the targeted Vt [35]. Thus, this mode behaves in a manner similar to the pressure control modes in terms of the pressure and flow patterns but delivers the predetermined Vt by adjusting the PIP on the basis of the lung compliance. This ventilation mode has proven to be very effective in VLBW infants demonstrating a smaller duration of mechanical ventilation and less hemodynamic perturbations [32, 33] as well as in infants after congenital heart surgery in whom intratracheal pressure was reduced and the hemodynamics were stable [34]. When this mode is combined with other ventilator options that allow the infant to breathe spontaneously with pressure support, it is called “automode,” which is now present on some anesthesia machines although again without studies in neonates.
High-Frequency Ventilation
High-frequency ventilation (HFV) has found application in the management of neonates with serious lung disease since it allows ventilation with small tidal volumes and a mean airway pressure (MAP) that is greater than that obtained with conventional ventilation. This strategy has been very effective in infants with severe respiratory failure since HFV improves the gas exchange by optimizing the lung volume while ventilating with small tidal volumes and reduced proximal airway pressures, thereby avoiding damage to the lungs [35]. HFV improves the gas exchange by enhancing both the convection and diffusion of the respiratory gases. The principle is based on the natural “resonant” frequency of the lung and the fact that less pressure is required to move the gas into and out of the lungs at this resonant frequency, which is approximately 10 Hz (1 Hz = 60 bpm) in neonates and even greater in premature infants than with conventional ventilation.
A number of different HFV ventilator strategies are available although, once again, no evidence distinguishes one strategy over another. The first strategy was high-frequency jet ventilation (HFJV), a very well-established technique in anesthesia that delivers short bursts of gas (with very short T i) at very high frequencies (up to 600/min) superimposed on a constant flow that determines the level of PEEP. This strategy requires a specific tracheal tube. HFJV has failed to prove its usefulness in clinical practice, with conflicting results on the neurological and respiratory outcomes in neonates [36–38]. Another strategy for the provision of HFV is high-frequency flow interruption (HFFI), which consists of a continuous gas flow delivered by a high-pressure gas source that is interrupted at a high frequency (up to 20 Hz) [39]. However, this technique failed to demonstrate any beneficial advantage in terms of the pulmonary outcome, and some studies have even identified a greater incidence of air leaks in premature infants treated with HFFI [40, 41]. Currently, the most frequently used modality is high-frequency oscillatory ventilation (HFOV). This strategy is based on the presence of an electromagnetically driven piston or vibrating diaphragm that generates biphasic pressure waveforms at very high frequency (up to 15 Hz). HFOV has both an active inspiratory phase and an active expiratory phase (by inducing a negative proximal airway pressure during exhalation). With HFOV, the I/E ratio is adjusted to avoid gas trapping that results from inadequate time for exhalation [21]. HFOV provides very small oscillatory tidal volumes that are superimposed on an adjustable MAP. Theoretically, HFOV is particularly beneficial when an increased volume strategy is required to maintain FRC, as HFOV maintains FRC with a reduced MAP compared with other modes of ventilation. When HFOV is applied to premature infants with chronic lung disease, the oscillatory volumes are determined by the position of the tidal volume on the pressure–volume curve and the pressure amplitude [42]. It may offer some advantages as a ventilator strategy in neonates with congenital diaphragmatic hernia or severe respiratory distress with markedly reduced lung compliance. Nonetheless, a recent meta-analysis failed to demonstrate evidence that HFOV offers any advantages over conventional ventilation, when used as a primary or rescue mode to ventilate infants with an acute pulmonary dysfunction [43–45]. HFOV may be associated with a reduced incidence of chronic lung disease in premature infants [44, 46], but this requires confirmation.
Continuous Positive Airway Pressure and Noninvasive Ventilation
A large number of neonates benefit from a noninvasive respiratory support that allows the application of continuous positive airway pressure (CPAP) and/or the delivery of noninvasive ventilation (NIV). The aim of nasal CPAP (nCPAP) is to maintain FRC by recruiting and maintaining airway patency and lung expansion [47, 48]. It also decreases the WOB and reduces the frequency of apnea of prematurity [49]. As a result, it is routinely used in clinical practice to support the lungs in preterm infants whose airways were recently extubated and as an alternative to tracheal intubation and ventilation, to support those experiencing significant apnea of prematurity and those with respiratory distress soon after birth. Some techniques also provide a phasic positive increase in pressure (pressure support or pressure controlled) in addition to nCPAP that can be synchronized (SNIMV, synchronized nasal intermittent mandatory ventilation) or not (NIMV) to the infant’s respiratory efforts [48]. This is also known as nasal intermittent positive-pressure ventilation (NIPPV) [49, 50]. During the past decade, the use of NIV for acute respiratory failure in neonates in NICUs has been expanding. Predictive factors for the successful use of NIV have recently been identified [51]. Meta-analyses have failed to demonstrate the benefit of NIV in the presence of respiratory distress syndrome [52], although it does prevent extubation failures in neonates [53, 48]. Accordingly in preterm infants, NIV reduces the need for reintubation, invasive mechanical ventilation, and surfactant during the first 72 h after birth [47, 50, 54–56]. For this purpose, NIV is often started after the minimal PEEP level is set to ~6 cm H2O and the PIP to between 10 and 12 cm H2O.
nCPAP can be delivered by two means: (1) a continuous flow and a device that varies exhalation either by modifying the expiratory orifice size or (2) by immersing the distal end under water to a depth that determines the level of CPAP. This latter is also termed bubble nCPAP. The bubbles create pressure oscillations that are transmitted to the airway. It has been suggested that this phenomenon may improve gas exchange by facilitating gas diffusion [57]. Evidence indicates that the regional distribution of ventilation with bubble nCPAP depends solely on anatomical ventilator patterns and is independent of the infant’s position [58, 2]. A variable-flow mode that modifies the nCPAP uses nasal prongs that redirect the exhaled gas out the expiratory limb. The WOB with the variable-flow nCPAP is less than that with the bubble nCPAP [59]. Another approach that is based on the variable-flow setting is the bi-level nCPAP or BiPAP. BiPAP allows the child to trigger inspiration and to breathe between two levels of positive pressure, with some systems including an abdominal wall sensor to help synchronize the delivery of positive pressure with the child’s inspiratory efforts. The BiPAP system appears to be superior to the nCPAP system in improving oxygenation and ventilation in LBW infants [60, 61].
The application and success of nCPAP and NIV rely on the airway interfaces and their ability to guarantee comfort and optimize the delivery of pressure. Of all the interfaces available to provide nCPAP, the binasal prongs appear to be the optimal design [62]. These nasal prongs are tight fitting in the nostrils, preventing an air leak. While prongs may be associated with a high incidence of nasal trauma in infants [21], leaks remain a major concern in NIV. Such leaks may reduce alveolar ventilation, lead to child-ventilator asynchrony, and increase nasal resistance. Nonetheless, when nCPAP is applied soon after birth, it may reduce the need for surfactant, the frequency of chronic lung disease, and sequelae including death in preterm infants [63].
High-flow nasal cannula (HFNC) (defined as an oxygen/air mixture flow rate >1 l/min) is used in preterm infants to support pulmonary function as well as deliver increased oxygen concentrations. The gas flow may or may not be heated and humidified [48]. The heated and humidified modes are commonly used to minimize nasal mucosal trauma, although unheated HFNC has also been used successfully. HFNC has found favor in preterm infants with apnea, chronic lung disease, and respiratory distress syndrome. This strategy may be effective as it flushes gas from the dead space of the nasopharyngeal cavity, facilitates improved alveolar ventilation, and promotes more efficient respiration [48]. In contrast to the nasal cannula used in nCPAP, these cannulas do not fit into the nasal passages snugly but have a large air leak. Although the presence of a leak was initially thought to reduce the incidence of nasal injury, nasal mucosal injury, nasal obstruction or bleeding, and even nosocomial infection have been reported [64, 65]. However, after tracheal extubation, HFNC may be associated with a greater frequency of tracheal intubation than nasal CPAP [55]. A recent review concluded there was insufficient evidence to determine whether HFNC is effective in preterm infants [64, 65].
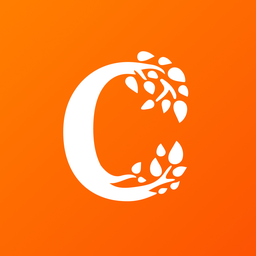
Full access? Get Clinical Tree
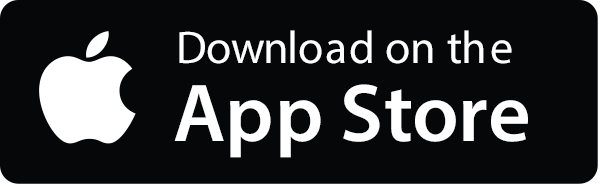
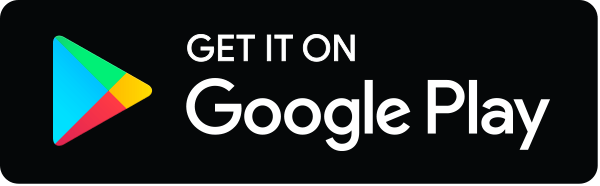