Neonatal Anesthesia
Steven C. Hall
Santhanam Suresh
Key Points








Related Matter
Inhalation Induction
Physiology of the Infant and the Transition Period
An infant’s first year of life is characterized by an almost miraculous growth in size and maturity. The body weight alone changes by a factor of three, and there is no other period in extrauterine life when changes occur so rapidly. Before birth, fetal growth and development depend on the genetic composition of the fetus, the mother’s placental function, and potential exposure to chemicals or infectious agents that can affect mother, fetus, or both. After birth, the newborn must rapidly adjust to the extrauterine environment to survive. The dramatic changes in functions of several systems will determine the viability of the neonate, as well as its ability to grow and develop properly.

The Cardiovascular System
Fetal Circulation
The fetal circulation is characterized by a parallel system in which both ventricles pump most of their output into the systemic circulation. Less than 10% of the combined cardiac output goes through the fetal pulmonary circulation via three main shunts; through the placenta, foramen ovale, and ductus arteriosus (Fig. 41-1A). The placenta provides oxygenated blood into the ductus venosus, the inferior vena cava, and then into the right atrium. In the right atrium, the majority of the oxygenated blood primarily flows through the foramen ovale into the left atrium,
bypassing the right ventricle and the pulmonary vascular bed. This preferential flow across the foramen occurs because of the relatively low pressure in the left atrium compared with that of the right atrium. Some blood from the right atrium does flow through the right ventricle and into the main pulmonary artery. The pulmonary vascular resistance is quite high in utero because of alveolar collapse and compression of blood vessels, inhibiting flow through the pulmonary circulation. The pulmonary vascular resistance is also high at this point because of the relatively low PaO2 and pH of the blood that does flow through the vessels. Some blood in the pulmonary artery does flow through the pulmonary circulation and then into the left atrium, but the majority of flow goes through the ductus arteriosus into the descending aorta.
bypassing the right ventricle and the pulmonary vascular bed. This preferential flow across the foramen occurs because of the relatively low pressure in the left atrium compared with that of the right atrium. Some blood from the right atrium does flow through the right ventricle and into the main pulmonary artery. The pulmonary vascular resistance is quite high in utero because of alveolar collapse and compression of blood vessels, inhibiting flow through the pulmonary circulation. The pulmonary vascular resistance is also high at this point because of the relatively low PaO2 and pH of the blood that does flow through the vessels. Some blood in the pulmonary artery does flow through the pulmonary circulation and then into the left atrium, but the majority of flow goes through the ductus arteriosus into the descending aorta.
Changes at Birth
After birth, all of these shunts are eliminated or start to close quickly.1 The placental shunt is eliminated and the ductus venosus is closed. The newborn’s left ventricle is now pumping blood into the higher pressure systemic circulation exclusively. Expansion of the lungs and initiation of breathing lead to dramatic changes in both the circulatory and pulmonary systems (Fig. 41-1B). As alveoli fill with air, the compression of the pulmonary alveolar capillaries is relieved, reducing pulmonary vascular resistance and promoting flow through the pulmonary circulation. This blood is now oxygenated, raising the arterial PO2, and further reducing pulmonary vascular resistance. Although the change in the first minutes to hours is dramatic, it usually takes 3 to 4 days for the pulmonary vascular resistance to decrease to normal levels. The foramen ovale will usually functionally close in the first hour of life as the increase in left atrial pressure from increased pulmonary circulation after the initiation of breathing exceeds right atrial pressure. The foramen is closed by a flap of tissue that covers the foramen. This foramen can reopen if there is a relative increase in right atrial pressure such as is seen with elevated pulmonary vascular resistance or fluid overload. Anatomic closure usually occurs in the first year of life, but may remain probe-patent into adulthood in 10% to 20% of patients. The ductus arteriosus starts to close in the first day of life and is usually functionally closed in the second day of life. In utero, patency of the ductus was determined by the combined relaxant effects of low oxygen tension and endogenously produced prostaglandins, especially prostaglandin E2. In a full-term neonate, oxygen is the most important factor controlling ductal closure. When the Pao2 of blood in the ductus rises to about 50 mm Hg, the muscle in the vessel constricts. It should be noted that the ductus of a preterm infant is less responsive to increased oxygen, even though its musculature is developed.
Myocardial function is different in the neonate. The neonatal cardiac myocyte has less organized contractile elements than the child or adult.2 Not only are there fewer myofibril elements, but they are not organized in parallel roles, as seen in the child and adult heart, making them less efficient. The neonate myocyte also has a less mature sarcoplasmic reticulum system. The underdeveloped sarcoplasmic reticulum is associated with a decrease in Ca2+-adenosine triphosphatase activity, an important component of contractility. As the sarcoplasmic reticulum matures, the efficiency of calcium transport and subsequent contractility increases.3 The neonatal myocardium cannot generate as much force as that of the older child and is relatively noncompliant. Consequently, there is limited functional reserve in the neonatal period, with afterload increases particularly poorly tolerated. After birth, there are dramatic changes in the myocardium. As the work of the ventricles increases secondary to high stroke volume and increased vascular resistance, these myocytes grow quickly in number and size. This growth is more dramatic in the left ventricle than the right ventricle because of the rise in systemic vascular resistance and fall in pulmonary vascular resistance, respectively.
Especially in the first 3 months of life, the parasympathetic nervous system influence on the heart is more mature than the sympathetic system and the myocardium does not respond to inotropic support as well as the older child or adult. There is animal evidence that there are maturational changes in β-adrenergic receptor function that explain the decreased responsiveness to inotropes in the neonate.4 The neonatal myocardium does have increased glycogen stores and higher rates of anaerobic glycolysis, which may explain its relative resistance to hypoxia and better performance in the presence of an ischemic insult. Because the myocardium is relatively noncompliant in the newborn, preload changes can increase stroke volume and cardiac output, but not as effectively as in the older child.5 In other words, the Frank–Starling relationship is present in the neonatal heart, but is not as effective as in the adult. The other clinical implication of a noncompliant ventricle is that, in the absence of significant increases in stroke volume, cardiac output is not well maintained in the presence of bradycardia. Lastly, neonates have immature baroreceptors. The baroreceptor is responsible for the reflex tachycardia that occurs in response to hypotension. Therefore, the immaturity of this reflex would limit the neonate’s ability to compensate for hypotension. In addition, the baroresponse of the neonate is more depressed than that of the adult at the same level of anesthesia.
In summary, the neonatal heart has some significant limitations. The resting cardiac output is much higher relative to body weight than in the adult because of the higher O2 consumption per kilogram of body weight. Stimulation of the myocardium produces a limited increase in contractility and cardiac output. The sympathetic nervous system, which usually provides the important chronotropic and inotropic support to the mature circulation during stress, is severely limited in the neonate because of lack of development. Even in the absence of stress, the neonatal heart has limited ability to increase cardiac output compared with the mature heart (Fig. 41-2). The resting cardiac output of the immature heart is close to the maximal cardiac output, so there is a limited reserve. The mature heart can increase cardiac output by 300%, whereas the immature heart can only increase cardiac output by 30% to 40%.
The Pulmonary System
The pulmonary system develops rapidly during the last trimester, with important changes in both the number of alveoli and the maturity of the pulmonary vascular system.6 These systems have not matured enough to provide adequate gas exchange until about 24 to 26 weeks’ gestation. The airways and alveoli continue to grow after birth, with alveoli increasing in number until about 8 years of age.7 With the initiation of ventilation, the pulmonary system changes dramatically. The alveoli transition from a fluid-filled to an air-filled state and a normal ventilatory pattern with normal volumes develops in the first 5 to 10 minutes of life. In order to adequately expand the collapsed and fluid-filled alveoli, the newborn will generate an initial negative intrathoracic pressure in the range of 40 to 60 cm H2O. By 10 to 20 minutes of life, the newborn has achieved its near-normal functional residual capacity (FRC), and the blood gases stabilize with the establishment of increased pulmonary blood flow. Table 41-1 lists the normal blood gases for the various periods of life.
The initial breaths to expand the lungs and subsequently maintain FRC are necessary components of the stabilization of the ventilatory system, as well as the circulatory system. Failure to do so will quickly lead to deterioration of both systems.
The initial breaths to expand the lungs and subsequently maintain FRC are necessary components of the stabilization of the ventilatory system, as well as the circulatory system. Failure to do so will quickly lead to deterioration of both systems.

Lung compliance is relatively low, but chest wall compliance is relatively high, compared to that of older children. The pliable rib cage gives less mechanical support than in the older child, leading to significant retractions with less efficient gas exchange and functional airway closure, thus increasing the work of breathing. The intercostal muscles are poorly developed at birth, with the diaphragm providing most of the gas exchange. The diaphragm
in the neonate has two types of fibers, the type 1, slow twitch, high-oxidative fibers that give sustained contraction with very little fatigue, and the type 2, fast twitch, low-oxidative fibers that give quick contractions, but fatigue easily. The distribution of these fibers in the newborn shows only about 25% type 1 fibers, while 55% of the fibers are type 1 in the mature diaphragm at about 2 years of age. The preterm newborn has even fewer type 1 fibers at birth, in the 10% range. This relative lack of type 1 fibers means that the newborn, especially the preterm, is at risk for diaphragmatic fatigue in the presence of significant resistance to ventilation or periods of hyperventilation.
in the neonate has two types of fibers, the type 1, slow twitch, high-oxidative fibers that give sustained contraction with very little fatigue, and the type 2, fast twitch, low-oxidative fibers that give quick contractions, but fatigue easily. The distribution of these fibers in the newborn shows only about 25% type 1 fibers, while 55% of the fibers are type 1 in the mature diaphragm at about 2 years of age. The preterm newborn has even fewer type 1 fibers at birth, in the 10% range. This relative lack of type 1 fibers means that the newborn, especially the preterm, is at risk for diaphragmatic fatigue in the presence of significant resistance to ventilation or periods of hyperventilation.
Table 41-1. Normal Blood Gas Values in the Neonate | |||||||||||||||||||||||||||||||||||
---|---|---|---|---|---|---|---|---|---|---|---|---|---|---|---|---|---|---|---|---|---|---|---|---|---|---|---|---|---|---|---|---|---|---|---|
|
Table 41-2. Comparison of Normal Respiratory Values in Infants and Adults | |||||||||||||||||||||
---|---|---|---|---|---|---|---|---|---|---|---|---|---|---|---|---|---|---|---|---|---|
|
Lastly, the continued presence of surfactant is necessary to maintain both the distensibility of the alveoli and the maintenance of an FRC at exhalation. Decreased surfactant production, due to prematurity or other conditions such as maternal diabetes, can cause respiratory distress syndrome (RDS). The decreased surfactant can cause alveolar collapse, decrease in lung compliance, hypoxia, increased work of breathing, and respiratory failure.8 Commercially available surfactant is extraordinarily useful to both treat and prevent RDS in susceptible patients. In addition, surfactant can improve gas exchange in preterms who may not have RDS, but are stressed by sepsis, heart failure, or other systemic problems.9 Delivered through an endotracheal tube, it can be used prophylactically in the very preterm newborn to prevent RDS, as well as treat newborns who have developed RDS.
In addition to the mechanical aspects of the pulmonary system, control of breathing has unique aspects in the neonatal period, especially in the preterm. Neonates respond less to hypercapnia than the older child. In addition, neonates respond to hypoxia with a brief period of hyperventilation, followed by hypoventilation. The initial hyperventilatory response can be prevented by hypothermia. Lastly, a periodic breathing pattern is common in neonates, especially in preterm newborns, that can persist up to a year of age.
Persistent Pulmonary Hypertension of the Newborn

Meconium Aspiration
Another important pulmonary issue in the newborn period is meconium aspiration. Interference with the normal maternal placental circulation in the third trimester may cause fetal hypoxia. Fetal hypoxia can result in an increase in the amount of muscle in the blood vessels of the distal respiratory units. Figure 41-5
illustrates the muscle increase found in blood vessels of a series of 11 infants who died of persistent pulmonary hypertension.12 Chronic fetal hypoxia leads to the passage of meconium in utero. The fetus breathes in utero so the meconium mixed with amniotic fluid enters the pulmonary system. Meconium aspiration can be a marker of chronic fetal hypoxia in the third trimester. This condition is different from the meconium aspiration that occurs during delivery. This meconium at birth is thick and tenacious, and mechanically obstructs the tracheobronchial system. Meconium aspiration syndrome leads to varying degrees of respiratory failure, which can be fatal in spite of all treatment modalities.
illustrates the muscle increase found in blood vessels of a series of 11 infants who died of persistent pulmonary hypertension.12 Chronic fetal hypoxia leads to the passage of meconium in utero. The fetus breathes in utero so the meconium mixed with amniotic fluid enters the pulmonary system. Meconium aspiration can be a marker of chronic fetal hypoxia in the third trimester. This condition is different from the meconium aspiration that occurs during delivery. This meconium at birth is thick and tenacious, and mechanically obstructs the tracheobronchial system. Meconium aspiration syndrome leads to varying degrees of respiratory failure, which can be fatal in spite of all treatment modalities.
Current recommendations for intubation and suctioning for newborns at delivery with frank meconium aspiration or meconium staining (approximately 10% of newborns) emphasize a conservative approach.13 Routine oropharyngeal suctioning of meconium is recommended immediately at the time of delivery, but tracheal intubation and suctioning should be performed selectively. If the newborn is vigorous and crying, no further suctioning is needed. If meconium is present and the newborn is depressed, the trachea should be intubated and meconium and other aspirated material suctioned from beneath the glottis. If meconium is retrieved and no bradycardia is present, reintubate and suction. If there is bradycardia, administer positive pressure ventilation and consider suctioning again later once the neonate is stabilized.
The Renal System
In utero, most of the fetal waste material is removed by the maternal placenta. In effect, the fetal kidneys are passive, receiving relatively little renal blood flow and having a low glomerular filtration rate (GFR).14 There are four major reasons for the low renal blood flow and GFR: Low systemic arterial pressure, high renal vascular resistance, low permeability of the glomerular capillaries, and the small size and number of glomeruli. In utero, the kidneys receive only about 3% of the cardiac output, whereas they will receive about 25% of cardiac output in adulthood. At birth, this changes dramatically. The systemic arterial pressure increases and the renal vascular resistance decreases, and the kidneys now receive a progressively increased part of the cardiac output. At birth, the GFR is low, but increases significantly in the first few days, doubles in the first 2 weeks, but does not reach adult levels until about 2 years of age. The limited ability of the newborn’s kidney to concentrate or dilute urine results from this low GFR and decreased tubular function. However, during the first 3 to 4 days, the circulatory changes increase renal blood flow and GFR and improve the neonate’s ability to concentrate and dilute the urine. Part of the improvement in renal function is the establishment of gradients in the medullary interstitium that promotes resorption of sodium. The maturation continues, and by the time the normal full-term infant is 1 month of age, the kidneys are approximately 60% mature. Urine output is low in the first 24 hours, but then increases to an expected level of at least 1 to 2 mL/kg/hr. Diuresis after the first day of life <1 mL/kg/hr should be considered indicative of either hypovolemia or decreased renal function for another reason.
Despite the rapid maturation of renal function and the increased capacity of the neonatal kidneys, they still have limitations.15 From an anesthetic standpoint, the half-life of medications excreted by means of glomerular filtration will be prolonged.16 The relative inability to conserve water means that neonates, especially in the first week of life, tolerate fluid restriction poorly. In addition, the inability to excrete large amounts of water means the newborn tolerates fluid overload poorly. The newborn kidney is better able to conserve sodium than excrete sodium, making hypernatremia a risk if excess sodium is administered. However, because of the lack of tonicity in the medullary interstitium shortly after birth, there will be some obligate sodium loss in the first days of life. This improves as the countercurrent multiplier is developed in the interstitium.
Fluid and Electrolyte Therapy in the Neonate
Total body water (TBW), which is usually described in terms of percent of body weight, varies by both age and gestational status. The highest TBW is found in the fetus, but decreases to about 75% of body weight for a term infant at birth. Preterm infants have a higher TBW than term infants, often in the 80% to 85% range. TBW decreases during the first 12 months of life to about 60% to 65% of body weight and stays at this level through childhood.
TBW is distributed between two compartments, intracellular fluid (ICF) and extracellular fluid (ECF). The ECF volume is larger than the ICF volume in the fetus and newborn, usually in the 40% (ECF) and 20% (ICF) of body weight ranges. This is the opposite of the situation in infants and children. There is a significant diuresis after birth that produces a decrease in the ECF volume. In addition, ICF volume increases because of the growth of cells in the body. The ECF/ICF volumes (20% and 40% of body weight) approach adult values by about 1 year of age.
This dramatic shift is beneficial to the child, especially in increasing the mobility of reserves in the face of dehydration. Fluid can be easily mobilized from ICF volumes to replenish intravascular volume that is lost from fasting, fever, diarrhea, or other causes. This means that the nonneonate is better situated to maintain intravascular volume in these situations than the neonate.
This dramatic shift is beneficial to the child, especially in increasing the mobility of reserves in the face of dehydration. Fluid can be easily mobilized from ICF volumes to replenish intravascular volume that is lost from fasting, fever, diarrhea, or other causes. This means that the nonneonate is better situated to maintain intravascular volume in these situations than the neonate.
The ECF is divided into the plasma and the interstitial fluid. The plasma water is usually about 5% of body weight and the related blood volume, assuming a hematocrit of 45%, is about 8% of body weight in infants and children. The water content is slightly higher in neonates and may approach 10% of body weight in preterms. The interstitial fluid, usually about 15% of body weight, can demonstrate large increases in disease states associated with liver failure, heart failure, renal failure, and other causes of conditions such as pleural effusions or ascites. The reason for this is the balance between oncotic and hydrostatic forces. Any condition that decreases oncotic pressure, such as loss of albumin in liver failure, promotes the loss of fluid into the interstitial fluid. On the other hand, raised hydrostatic pressures, such as seen in heart failure, can result in fluid leaving the plasma and accumulating in the interstitial space. Conditions that result in translocation of fluid from the plasma to the interstitial spaces, whether because of decreased oncotic pressure or increased oncotic pressure, are of significant consequence to the neonate. Loss of fluid from the plasma volume compromises the intravascular volume, potentially decreasing the perfusion of vital organs and systems.
The blood volume in the normal full-term newborn is approximately 85 mL/kg and approximately 90 to 100 mL/kg in the preterm, although estimates of these volumes can vary between studies. Approximately 50 mL/kg of this volume is the plasma volume. For all practical purposes, the electrolyte values in the neonatal period are the same as in the child and adult with the exception of potassium, which can be about 1 to 2 mmol/L higher than average for the first 2 days of life.17
Maintenance fluid requirements increase during the first days of life. They have been estimated to be 60, 80, 100, and 120 mL/kg/24 hr for the first 4 days of life, respectively. For the rest of the neonatal period, a maintenance rate of 150 mL/kg/24 hr is appropriate.
The appropriate type of maintenance fluid depends on several issues. Because of ongoing sodium loss secondary to the inability of the neonatal distal tubule to respond fully to aldosterone, intravenous fluids in the neonate must contain some sodium. Most operations on neonates involve loss of blood and ECF, which must be replaced with a fluid of similar electrolyte content (i.e., a balanced salt solution such as lactated Ringer or Plasma-Lyte). Hypotonic solutions should not be used to replace these losses because they can cause significant hyponatremia. Thus, if the neonate is already stable on a maintenance solution, it is reasonable to continue this maintenance at a constant rate, adding balanced salt solution or colloid or blood products as needed.
The other issues for fluid choice in the neonate center on appropriate glucose administration. In most cases, maintenance fluids containing 10% glucose and 0.2 normal saline with 20 mmol/L of potassium are reasonable in the first 48 hours of life. Beyond that time period, full-term infants may do well with 5% glucose instead of 10%, although preterms will often require the higher glucose load longer. Newborns of diabetic mothers, those who are small for gestational age, and those who have had continuous glucose infusions stopped have particular problems with hypoglycemia. These infants need to have their blood glucose values monitored. Neonates who are scheduled for surgery and have been receiving hyperalimentation fluids or supplementary glucose must continue to receive that fluid during surgery or must have their glucose levels monitored because of concerns of hypoglycemia. There is little consensus on the issue of what constitutes hypoglycemia.18
The concern about hypoglycemia must be balanced against the potential augmentation of ischemic injury by the administration of glucose leading to hyperglycemia. Interestingly, there are observational reports examining neonates undergoing cardiac surgery in which high glucose concentrations during or after the surgery were not associated with worse neurodevelopmental outcomes.19,20 These studies support the contention that avoiding hypoglycemia may be preferable to restricting glucose in newborns and risking hypoglycemia, at least in those having cardiac surgery.
Blood Component Therapy in the Neonate
Most of the basic principles of blood component therapy are the same in newborns and older children and adults. The first principle is to ensure adequate circulating intravascular volume and add components, as needed. However, there are a few important differences. These differences are related to the interconnection of maternal and fetal blood circulations and the flow of some, but not all elements, across the placenta, the incompletely developed immune system of the neonate, and the small blood volume of the neonate. The indications in the perioperative period for red blood cells are similar to those for adults, but the target values in available guidelines are higher.21 Transfusion is indicated for a hemoglobin <10 g/dL for major surgery or in a newborn with moderate cardiopulmonary disease, while transfusion for a hemoglobin <13 g/dL is indicated in a newborn with severe cardiopulmonary disease. It is also recommended that platelets be kept above 50,000 for invasive procedures. These recommendations are based on expert consensus, not prospective studies.
The hemoglobin in transfused blood is hemoglobin A, as opposed to the hemoglobin F in the neonate at birth. An advantage of the transfused blood is better release of oxygen at the tissue level from hemoglobin A. Fresh blood cells have the advantage of lower potassium levels, especially during rapid transfusion, than older blood, although washed or frozen cells prevent this problem. Transfusion-associated graft-versus-host disease is a rare but potentially deadly complication of red blood cell transfusion. Transfused lymphocytes in the donor blood attack the recipient bone marrow and other tissues, causing fever, pancytopenia, diarrhea, and hepatitis. To prevent this, gamma irradiation of cellular blood components is used to destroy lymphocytes and prevent transfusion-associated graft-versus-host disease. For this reason, irradiated blood is routinely used for transfusion of preterm newborns and, in many centers, for all newborns under 6 months of age. Leukoreduction by filtration is also used to reduce cytomegalic virus transmission. Lastly, because there is very weak expression of the ABO antigens at birth, ABO typing, Rh typing, and an initial antibody screen are commonly done, although cross-matching is not.
The Hepatic System
The functional capacity of the liver is immature in the newborn, especially synthetic and metabolic functions. Although most enzyme systems for both normal function and drug metabolism are present at birth, the systems have not yet been induced.22 In utero, the maternal circulation and metabolism were responsible for the majority of elimination of drugs. As the newborn develops, the different hepatic metabolic pathways mature at different rates. Conjugation by sulfation and acetylation are relatively well
developed in the newborn, with conjugation with glutathione and glucuronidation less well-developed.23 Some of these pathways do not achieve adult levels of activity until after 1 year of age.24,25 Because of this immaturity, some drugs that undergo hepatic biotransformation, such as morphine, have prolonged elimination half-lives in newborns.26 Other drugs, such as lidocaine, do not undergo prolonged elimination in the newborn. In some drugs, such as caffeine, the lack of hepatic metabolism of the drug is balanced by excretion of an increased amount of unchanged drug through the kidney. Up to 85% of unmetabolized caffeine may be found in the urine in the newborn, compared with 1% in the adult.27
developed in the newborn, with conjugation with glutathione and glucuronidation less well-developed.23 Some of these pathways do not achieve adult levels of activity until after 1 year of age.24,25 Because of this immaturity, some drugs that undergo hepatic biotransformation, such as morphine, have prolonged elimination half-lives in newborns.26 Other drugs, such as lidocaine, do not undergo prolonged elimination in the newborn. In some drugs, such as caffeine, the lack of hepatic metabolism of the drug is balanced by excretion of an increased amount of unchanged drug through the kidney. Up to 85% of unmetabolized caffeine may be found in the urine in the newborn, compared with 1% in the adult.27
Lastly, decreased metabolism of a drug may actually increase its safety profile. Acetaminophen undergoes less biotransformation by the cytochrome P450 system in the newborn, producing less reactive metabolites that are toxic. Paradoxically, neonates can tolerate dosages of acetaminophen that would be hepatotoxic in adults.28,29 Synthetic function of the liver is also altered in the neonatal period. Levels of albumin and other proteins necessary for binding of drugs are low in term newborns (and are even lower in preterm infants) and impacts the ability to bind drugs, producing greater levels of free drug. This phenomenon is especially true for the binding of alkaline drugs that bind to α1—acid glycoprotein such as synthetic opioids and local anesthetics. The ability to bind to existing albumin may also be altered by hyperbilirubinemia for some medications. The need for exogenous vitamin K in the newborn is another consequence of this decrease ability. Because of decreased synthetic function, neonatal hepatic glycogen stores are low, especially in the preterm, increasing the risk of hypoglycemia in response to stress.
Anatomy of the Neonatal Airway

![]() Figure 41.6. Complicating anatomic factors in infants. (Modified from: Smith RM. Anesthesia for Infants and Children, 4th ed. St Louis: Mosby; 1980:16, with permission.) |
The subglottic area is funnel-shaped, unlike the adult airway (Fig. 41-7). In adults, the narrowest aspect of the upper airway is at the vocal cords, but in the neonate there is further narrowing until the level of the cricoid ring, the first complete cartilaginous ring. Because this narrowing is susceptible to trauma from intubation or too large an endotracheal tube, uncuffed tubes have traditionally been used in the neonatal period, although cuffed tubes are increasingly popular beyond the first few months of life. The use of cuffed, small volume, high resistance endotracheal tubes have been demonstrated to provide an adequate airway with marginal changes to the diameter of the airway leading most practitioners
to now use a cuffed tube in even neonates and young infants.32 A narrow cricoid ring is significant because it means that the narrowest portion of the neonate’s airway is not the vocal cords but the cricoid ring, this has to be kept in mind especially if an attempt to push an endotracheal tube after entrance to the glottis is considered.
to now use a cuffed tube in even neonates and young infants.32 A narrow cricoid ring is significant because it means that the narrowest portion of the neonate’s airway is not the vocal cords but the cricoid ring, this has to be kept in mind especially if an attempt to push an endotracheal tube after entrance to the glottis is considered.
Finally, the infant has a large occiput so the head flexes forward onto the chest when the infant is lying supine with his head in the midline. Further flexion of the neck can cause obstruction. Extreme extension can also obstruct the airway, so a midposition of the head with slight extension is preferred for airway maintenance. Rarely, this may require placing a small roll at the base of the neck and shoulders.
Anesthetic Drugs in Neonates

Volume of distribution. Water is predominantly in greater proportion in premature and full-term infants,33 which increases the need for larger doses of medications that are water-soluble.
Protein binding. Neonates have decreased protein and hence have a decrease in protein binding of most drugs. This leads to increased free drug levels, which leads to increased toxicity of drugs that are predominantly protein bound.34
Fat content. Neonates have a decreased amount of fat and muscle mass, which leads to greater levels of drugs that are primarily redistributed to muscle and fat. Decreased renal and hepatic function predisposes neonates to increased blood levels from normal doses that are used for induction and maintenance of anesthesia.
Intravenous Agents
Anticholinergics
Anticholinergics such as atropine and glycopyrrolate are used frequently in neonates. They may be helpful in decreasing secretions and decreasing the response to vagal stimulation on intubation. The dose of atropine is 10 μg/kg given intravenously and 20 μg/kg if given intramuscularly. It may be desirable intramuscularly in certain situations prior to induction of anesthesia, especially in emergency surgeries. Caution should be exercised if neonates have other associated congenital abnormalities, particularly narrow angle glaucoma in which case it could increase intraocular pressure. Glycopyrrolate, a synthetic quaternary ammonium compound, has a longer duration of action than atropine and may potentially have less central effects because of decreased penetration of the blood–brain barrier.
Midazolam
This is a water-soluble benzodiazepine that can be used for premedicating infants prior to surgery. Clearance of midazolam is lower in neonates and premature infants, and hence caution has to be exercised with the amount of midazolam used. If combined with opioids, intravenous midazolam can cause severe hypotension. A common modality for midazolam administration in neonatal intensive care units is by continuous infusion. If a patient is receiving midazolam infusion, care should be taken to avoid large doses of opioids. Our recommendation is to stop midazolam during surgery, although it is recommended to resume the infusion after surgery to avoid withdrawal symptoms.
Sedative/Hypnotics
The common sedative/hypnotics used in neonates include propofol, thiopental, and ketamine.
Thiopental
Because of the large volume of distribution in neonates, it may be necessary to use large doses of thiopental for induction of anesthesia. There has been an acute shortage of thiopental for induction of anesthesia and hence is now rarely used in North America. However, because of their reduced clearance, the effect may last longer than anticipated. Thiopental can cause hypotension in neonates who are volume depleted, especially in infants who are scheduled for emergency surgery. It should be avoided in neonates with congenital heart disease because of its effect on myocardial function leading to hypotension. A dose of 2 to 4 mg/kg is usually well tolerated by most neonates for induction of anesthesia. When compared with intubation without any hypnotic, the use of intravenous thiopental demonstrated adequate maintenance of heart rate and blood pressure.35
Propofol
Propofol, a phenyl sedative/hypnotic, is a commonly used induction agent in infants and children. In a randomized trial comparing intravenous propofol with atropine, succinylcholine, and morphine, it was noted that propofol maintained adequate hemodynamics in neonates.36 There is variability in elimination of propofol in neonates and preterm infants with longer elimination times.37 Hence, while using propofol, it is important to reduce the dose to ensure early wake up and extubation. This is the most common intravenous induction agent that is used in the United States.
Ketamine
Ketamine, an N-methyl-D-aspartic acid (NMDA) antagonist, is used for induction of anesthesia in neonates who may have cardiovascular instability. An induction dose of 2 mg/kg intravenously with a higher dose of 4 to 5 mg/kg is used intramuscularly. Although it produces hemodynamic stability, it can cause an increase in oral secretions. Recently, there have been significant alterations in excitotoxic cells in the animal model when exposed to NMDA receptor antagonists like ketamine with resultant concern about potential neurodegenerative changes with their exposure.38,39 All of these experimental models were using very high doses of ketamine unlike what is routinely recommended for induction of anesthesia in neonates. In addition, there are also another set of experimental data in animal studies that demonstrated a beneficial effect of ketamine in an experimental pain model.40 Ketamine is still used frequently in neonates with congenital heart disease for induction of anesthesia.41
Opioids
Opioids are used extensively in the management of anesthesia in neonates. The advantage of using opioids is their ability to maintain cardiovascular stability during major surgery. The common opioids used in neonates include fentanyl, morphine, and remifentanil. Infants who are on long-term doses of opioids may
develop tolerance and may have to be placed on methadone, a longer-acting opioid.42
develop tolerance and may have to be placed on methadone, a longer-acting opioid.42
Fentanyl
This synthetic opioid is commonly used for sedation in the neonate in the intensive care unit as well as in the operating room. A dose of 2 to 4 μg/kg/hr can maintain hemodynamic stability in these infants during surgery. The pharmacokinetics of fentanyl have been well studied in newborns.43 The use of fentanyl in association with benzodiazepines may lead to hypotension and hemodynamic instability.44 Caution must be exercised when the combination is administered during the perioperative period. Fentanyl may result in respiratory depression even with small doses. Continuous infusions may predispose to respiratory depression more frequently than bolus doses.45 Chest wall rigidity and glottic rigidity has been described with fentanyl.46 Small doses, as little as 1 to 2 μg/kg, can result in significant chest wall rigidity, leading to desaturation and need for mechanical ventilation. There is no significant maturational change on the brain associated with fentanyl compared with morphine. Hence, the sensitivity to fentanyl will not significantly change as the infant matures significantly. Fentanyl still continues to be the mainstay in newborns for sedation and analgesia.
Morphine
The kinetics of morphine have been studied in newborns.47 Premature babies have been shown to have decreased clearance. Morphine clearance (range, 0.8 to 6.5 mL/min/kg) correlated significantly with gestational age (r = 0.60; p < 0.01) and birth weight (r = 0.55; p < 0.01).48 Because of decreased clearance, dosing in neonates, especially premature infants, should be adjusted to be provided every 6 hours to allow for clearance of the drug.47 Morphine is used frequently in the intensive care unit for postoperative pain control. Morphine infusions in the perioperative period have resulted in minor prolongation of postoperative ventilation. However, the incidence of apnea or hypotension was not observed in neonates despite prolonged morphine infusions after successful extubation.49 A large multicenter neonatal trial (NEOPAIN) demonstrated very little neurobehavioral changes associated with preemptive analgesia with morphine in neonates in the neonatal intensive care unit.50
Morphine is metabolized to morphine-3-glucoronide and morphine-6-glucoronide. Morphine-6-glucoronide predisposes to respiratory depression. The sensitivity to morphine-6-glucoronide increases with increase in age because of increased maturation of the neuronal receptors.51 A minority of infants who are scheduled for surgery may have been on ECMO. Kinetics of morphine have been carefully studied in neonates undergoing ECMO and do not show significant variability.52
Remifentanil
Remifentanil is an ultra short-acting opioid that is metabolized by nonspecific esterases in plasma and tissues and has a half-life of <10 minutes. The pharmacokinetics of remifentanil in neonates are similar to that of older children.53 Remifentanil is used for maintenance of anesthesia by avoiding volatile anesthetic agents. The use of remifentanil infusion facilitated tracheal extubation in infants in a randomized trial when compared with volatile agents.54
Methadone
Methadone is a long-acting opioid that is used in neonates and infants in neonatal intensive care units, particularly when withdrawal from opioids is suspected. The pharmacokinetics of methadone are being studied in neonates; however, published data is not available. The “black box” warning against the use in infants with prolonged QT intervals is real and infants on long-term methadone use should be carefully monitored with serial ECGs. However, it is used frequently in managing opioid tolerance.42
Neuromuscular Blocking Agents
Neuromuscular blocking agents (NMBAs) are frequently used during neonatal anesthesia to facilitate tracheal intubation, assist with controlled ventilation, relax abdominal musculature, and ensure immobility. Factors that influence the choice of agent include the time of onset, duration of action, cardiovascular effects, and mechanism of clearance/elimination.
Succinylcholine
Succinylcholine, the only depolarizing muscle relaxant available, has the most rapid onset time of all the NMBAs. Neonates and infants have a larger ECF volume, leading to a larger volume of distribution and an increased dose requirement compared with children and adults. Thus, the recommended intravenous dose of succinylcholine for neonates and infants is 3 mg/kg, compared with 2 mg/kg in children, with an onset time of 30 to 45 seconds and duration of 5 to 10 minutes. The recommended intramuscular dose of succinylcholine is 4 mg/kg, with an onset time of 3 to 4 minutes and duration of approximately 20 minutes. Caution should be exercised when administering a second dose of succinylcholine because this can lead to vagally mediated bradycardia or sinus arrest. Pretreatment with atropine is recommended.55
The more recent succinylcholine controversy has called into question the use of succinylcholine in boys younger than 8 years.56 The reports of hyperkalemia with cardiac arrest in such children with unrecognized muscular dystrophy have led some clinicians to take the position that succinylcholine should not be used routinely for this group of patients. The occurrence of this problem is somewhere in the range of 1 in 250,000 anesthetics, with a mortality rate of 50%. Although a concern in young children, it is not a problem in the neonatal period. Succinylcholine is still recommended in rapid-sequence situations, potential difficult airway, or if there are airway emergencies with progressive desaturation. When it is evident that a neonatal airway is obstructed by laryngospasm or other reason and no progress is made in ventilation, intramuscular or intravenous succinylcholine should be administered. Hyperkalemia can be recognized by peaked T waves. However, the clinician may not see this particular electrocardiographic change because it occurs 2 to 3 minutes after drug administration, when the anesthesiologist is attending to the airway. The hyperkalemia interferes with conduction, leading to a bradycardia and, if severe enough, cardiac arrest. The drug of first choice is intravenous calcium, 10 mg/kg. The use of sodium bicarbonate, 1 mEq/kg, to treat any metabolic acidosis that may occur with arrest is also believed to be useful because alkalosis decreases hyperkalemia. At the same time, the patient should be hyperventilated to reduce the CO2, thereby encouraging a respiratory alkalosis. If there is refractory hypotension, an option is to administer epinephrine 5 to 10 μg/kg. One of the actions of epinephrine is to stimulate the sodium–potassium pump and cause the potassium to re-enter the cell, thereby reducing the serum level. If there is no response at this dose level, it should be increased incrementally until there is a response. Lastly, magnesium has been described as a treatment for hyperkalemia because it also antagonizes the effects of hyperkalemia, as does calcium.
Nondepolarizing Agents
The neonate’s neuromuscular junction is more sensitive to nondepolarizing muscle relaxants, and the neonate has a larger volume of distribution because of a large ECF volume.57 These two effects tend to balance each other so, roughly speaking, the dose of a nondepolarizing muscle relaxant for an infant is similar to that for a child on a milligram per kilogram basis. The ongoing organ maturation, which continues during the neonatal period, has a tremendous impact on the metabolism and clearance of the nondepolarizing agents. As a result, there is considerable variability and unpredictability in the duration of action of these agents in the neonatal period. Dosing should be titrated to effect and, when possible, guided by monitoring neuromuscular function with a nerve stimulator.
Intermediate Nondepolarizing Agents
Rocuronium
Rocuronium appears to be the drug of choice among the intermediate-acting, nondepolarizing muscle relaxants for neonates. The intubating dose of rocuronium is 0.6 mg/kg. The length of action of rocuronium in the neonate is similar to that in the older infant or child following an equipotent dose.58 Smaller doses (0.45 mg/kg) have been demonstrated to provide adequate relaxation but predictable recovery in newborn infants. However, if a larger dose of rocuronium, 1 to 1.2 mg/kg, is administered to avoid using succinylcholine during a rapid-sequence induction, then rocuronium will be a relatively long-acting muscle relaxant. Rocuronium is metabolized by the liver; however, unlike vecuronium there are no active metabolites. Rocuronium has mild vagolytic properties and may slightly increase heart rate.
Vecuronium
Although vecuronium is considered an intermediate-acting muscle relaxant in children and adults, in infants younger than 1 year it is considered a long-acting muscle relaxant. The duration of action of vecuronium is approximately twice that observed in children because of liver immaturity.59 Vecuronium undergoes primarily hepatic metabolism with production of active metabolites that are dependent on renal excretion. The recommended dose of vecuronium is 0.1 to 0.15 mg/kg, with an onset time of 90 seconds and duration of action of 60 to 90 minutes in the neonate. Even with increased doses, vecuronium has no effect on the cardiovascular system.
Pancuronium
Pancuronium is a long-acting NMBA with a pharmacokinetic profile similar to vecuronium. The recommended dose of 0.1 to 0.15 mg/kg has an onset time of 120 seconds and duration of 60 to 75 minutes. Unlike vecuronium, however, pancuronium primarily undergoes renal excretion.60 Pancuronium has vagolytic and sympathomimetic actions that cause tachycardia and an increase in blood pressure.61 In a relatively normal neonate with a normal blood pressure and normal blood volume, the use of pancuronium may result in hypertension, which has the potential to increase blood loss and increase the risk of hemorrhage in the extremely premature neonate. The risk for prolonged neuromuscular blockade in neonates, especially with altered renal function, makes pancuronium less desirable in neonates and infants undergoing minor outpatient surgical procedures.62 The use of pancuronium for prolonged durations especially in the intensive care units can lead to muscle weakness. Prolonged use has also been associated with sensorineural hearing loss in infants.63
Reversal Agents
The unpredictable nature of the NMBAs in the neonatal population, as well as the inability to accurately assess neuromuscular function in many situations, necessitates reversal of all nondepolarizing NMBA in neonates. The two commonly used reversal agents are edrophonium and neostigmine. Edrophonium in a dose of 1 mg/kg achieves a 90% reversal of a neuromuscular block in 2 minutes, whereas neostigmine in a dose of 0.07 mg/kg requires 10 minutes for a 90% reversal of neuromuscular block. This difference in time to peak effect allows the anesthesiologist to decide which agent is needed. Anticholinergic drugs like atropine or glycopyrrolate is coadministered to decrease the incidence of bradycardia. Neostigmine is the most common agent used for reversal of nondepolarizing muscle relaxants in neonates. The advantages of edrophonium over neostigmine are a more rapid reversal and fewer muscarinic side effects.
Volatile Agents
Volatile agents are used for maintenance of anesthesia in the neonatal period. Although halothane was the most commonly used volatile agent for many years and had a reasonable safety profile, the introduction of sevoflurane has clearly made a difference to the use of volatile agents in neonates. Desflurane, another potent volatile agent has limited application in children. Isoflurane is used for maintenance of anesthesia for longer surgical procedures. A brief synopsis of halothane followed by a more detailed description of sevoflurane will be described in this chapter.
Halothane
Halothane is still commonly used in many parts of the world as the primary inhaled anesthetic, although it is not currently used in the United States. Its long history for induction of anesthesia and its ease of use still make it a desirable agent in children. Halothane has a weak muscle relaxant property, facilitating induction and intubation without the use of a muscle relaxant. Halothane is a potent bronchodilator and may reduce the airway reflexes associated with intubation. The use of high doses of halothane for procedures including bronchoscopic evaluation of the airway may lead to significant myocardial depression and pump failure.64 Infants <8 weeks old and with a history of RDS with longer period of preoperative fasting are prone to hypotension. Halothane also sensitizes the myocardium to cardiac dysrhythmias. Animal experiments demonstrate the increased sensitivity to epinephrine with halothane when compared with isoflurane or sevoflurane.65 Hence, when concurrent exogenous catecholamines are administered (including epinephrine in local anesthetic solution), careful attention to the maximum dose should be carefully monitored. With the advent of sevoflurane, the use of halothane has decreased significantly in North America.
Isoflurane
Isoflurane has become a common maintenance volatile agent in neonates and infants. Its pungent odor does not allow its use for mask induction. Isoflurane increases the heart rate and hence may predispose to cardiac dysrhythmias. It has a greater effect in potentiation of muscle relaxation and hence plays an important role as a maintenance anesthetic. It is important to remember that the dose of muscle relaxants has to be reduced when isoflurane anesthesia is used. The dose of rocuronium bromide may have to be reduced to 0.45 mg/kg compared with a normal maintenance dose of 0.6 mg/kg.58 Isoflurane has less myocardial depression when compared with halothane in neonates.66
Sevoflurane
This is the newer volatile agent that offers an advantage for rapid induction and rapid awakening. It has a less pungent smell than isoflurane. Its pharmacodynamics have been studied in neonates and children with a fairly reasonable safety profile.67 In children with congenial cardiac disease, it has been shown to produce fewer hemodynamic changes when compared with isoflurane.68 Although it produces less myocardial depression, it has a greater effect on respiratory depression compared with halothane. Minute ventilation and respiratory frequency were significantly lower during sevoflurane than halothane anesthesia (4.5 compared with 5.4 L/m2/min, and 37.5 compared with 46.7 breaths per minute, respectively, p < 0.05). There was also significantly less thoracoabdominal asynchrony during sevoflurane anesthesia.69
Desflurane
Desflurane was touted to be the best volatile agent in children because of its partition coefficient being close to that of nitrous oxide, thereby allowing a rapid uptake. However, the pungent nature of the drug has made it difficult to use it for induction of anesthesia.70 When compared with sevoflurane, infants who were preterm were noted to wake up sooner with desflurane, although there were no reductions in postoperative respiratory events.71
Local Anesthetic Solutions
Local anesthetic solutions are represented by two main classes, the amino-amides (amides) and the amino-esters (esters). The main difference between the two classes is that the amides undergo enzymatic degradation by the liver and the esters are hydrolyzed by plasma cholinesterases.72
Amides
These are commonly used local anesthetic solutions in neonates and infants. Local anesthetics used in common clinical practice belonging to this class include lidocaine, bupivacaine, ropivacaine and, more recently, levobupivacaine. The main characteristics differentiating these drugs are their speed of onset, duration of action, and potential for cardiac toxicity. The ability of neonatal liver enzymes to metabolize and their ability to oxidize and reduce these drugs are decreased when compared with adults.33,73 At approximately 3 months of age, the conjugation of these drugs in the liver reaches adult levels. Older children can also achieve higher levels of local anesthetic solution than adults because of alteration in pharmacokinetics of the drugs. Local anesthetic solution levels have been shown to be higher in children undergoing intercostal nerve blocks compared with adults.74 After caudal administration of local anesthetics, peak plasma level is obtained in children and adults in approximately 30 minutes.75 The steady-state volume of distribution (VdSS) for amides is increased in children compared with adults, although clearance (CL) is similar.75 Elimination half-life (t1/2) is related to the volume of distribution and clearance as follows: t1/2 = (0.693 × VdSS)/CL. This results in a larger VdSS and prolongation of the elimination half-life, especially if a continuous infusion is used. The systemic absorption of local anesthetics is often based on the site of injection. On a decreasing scale, the incidence of complications with local anesthetic solution injections decrease, with the highest concentrations seen in the intercostal area followed by the caudal space, the epidural space, and peripheral nerve blocks. With newer technique in regional anesthesia, including ultrasound guidance, the volume and dose of local anesthetic solution can be significantly reduced.76
Toxicity of Local Anesthetic Solutions
Local anesthetic toxicity includes cardiac toxicity, central nervous system (CNS) toxicity, local reactions, and allergic reactions. Amide local anesthetic solutions may have a greater cardiac depressing effect than ester local anesthetic solution. Common local anesthetic compounds used in the neonatal and infant period include amide local anesthetics such as lidocaine, bupivacaine, ropivacaine, and levobupivacaine, and ester local anesthetics such as chloroprocaine. A brief description of each of these agents in children is provided here.
Bupivacaine
Bupivacaine is the most commonly used local anesthetic solution in infants and children in North America. The pharmacokinetics and the pharmacodynamics have been well studied in infants and children.77 The concentration of the local anesthetic used depends on the site, the desired density of blockade (motor and sensory), postoperative “street readiness,” and the potential for cardiovascular and neurotoxicity. The concomitant use of other local anesthetics including infiltration anesthesia has to be taken into consideration before a total volume of local anesthetic solution is taken into consideration. This is especially true in neonatal surgery in which large quantities of local anesthetic solution can sometimes be injected for skin infiltration. If upper safe limits are likely to be approached, it is reasonable to avoid local anesthetic solution for infiltration and use a dilute epinephrine solution instead. The preferred concentration for peripheral nerve blockade is 0.25% bupivacaine or 0.2% ropivacaine, and the preferred concentration for single dose bolus doses is 0.25% or 0.125% solution of bupivacaine or 0.2% ropivacaine. When a continuous infusion is desired, a 0.1% or 0.125% solution of bupivacaine is preferred. In premature infants and in infants weighing under 1 kg, we prefer using 0.0625% bupivacaine, or in some cases bolus doses given every 12 hours. Although clear guidelines do not exist for local anesthetic solutions, a rough rule of thumb is to use 0.2 mg/kg/hr for continuous infusions of bupivacaine and 2 mg/kg for bolus doses.78
Metabolism
Bupivacaine is bound to α1-glycoprotein. This may be altered in the newborn period.79 It is a racemic mixture of the levo and dextro enantiomers. Although the levo enantiomer is the active form that provides the clinical effect of the local anesthetic solution, the dextro enantiomer is responsible for the adverse effects related to local anesthesia, including cardiac toxicity and neurotoxicity.
Toxicity
The major adverse effect of bupivacaine is toxicity related to the cardiovascular and the CNS. Local anesthetics have the ability to cross the blood–brain barrier and can cause alterations in the CNS functions. Continuous infusions in neonates can predispose them to CNS toxicity sooner than older infants.80 In pediatric patients, the incidence of cardiac toxicity occurs sooner than neurotoxicity,80 which may be partly because children may be anesthetized and devastating neurotoxicity may not be noticed until significant cardiac toxicity is seen. Manifestation of bupivacaine toxicity may also be affected by the concomitant use of volatile agents for general anesthesia.
Dosage
Bupivacaine can be used for most peripheral nerve blocks as well as for epidural and caudal infusions in infants and
children. The maximum dosage suggested for bolus injections in the caudal space or epidural space for older children is 4 mg/kg and 2 mg/kg for neonates and infants.78 Dosage recommendations for continuous infusions is 0.4 mg/kg/hr in older children and 0.2 mg/kg/hr in neonates and infants.22 The concentration of the solution used for peripheral nerve blocks is usually 0.25% or 0.5%, bearing in mind the ceiling limit for maximum dosage. An example of a continuous infusion in a 4-kg neonate will be 0.2 mg/kg/hr; this will be equivalent to 0.8 mL/hr of a 0.1% solution of bupivacaine (1 mg/mL of bupivacaine).
children. The maximum dosage suggested for bolus injections in the caudal space or epidural space for older children is 4 mg/kg and 2 mg/kg for neonates and infants.78 Dosage recommendations for continuous infusions is 0.4 mg/kg/hr in older children and 0.2 mg/kg/hr in neonates and infants.22 The concentration of the solution used for peripheral nerve blocks is usually 0.25% or 0.5%, bearing in mind the ceiling limit for maximum dosage. An example of a continuous infusion in a 4-kg neonate will be 0.2 mg/kg/hr; this will be equivalent to 0.8 mL/hr of a 0.1% solution of bupivacaine (1 mg/mL of bupivacaine).
Ropivacaine
Ropivacaine is a newer amide local anesthetic. It is a levo enantiomer with relatively less cardiovascular and CNS side effects compared with bupivacaine.81 The pharmacokinetics of ropivacaine are such that caudal blocks with ropivacaine (2 mg/kg) in children (aged 1 to 8 years) result in plasma concentrations of ropivacaine well below toxic levels in adults.81 This dose was also noted to produce less motor block, but provide adequate analgesia. Mean maximum plasma concentration of total ropivacaine at 2 mg/kg was 0.47 mg/L. A threshold of CNS toxicity was noted at a plasma concentration of 0.6 mg/L. Body weight-adjusted clearance was the same as in adults (5 mL/min/kg). Ropivacaine clearance depends on the unbound fraction of ropivacaine rather than the liver blood flow.
Toxicity
Although the safety of ropivacaine has been demonstrated in animal experiments, there have been reports of CNS toxicity and cardiac toxicity associated with the use of epidural ropivacaine. It is important to understand that an overdose of ropivacaine can cause toxicity, making close attention to dosage as important with ropivacaine as with other local anesthetics. Our recommended dose is bolus dose of 2 mg/kg and an infusion rate of 0.2 mg/kg/hr.
Levobupivacaine
Levobupivacaine is a newer levo enantiomer that has fewer adverse effects than bupivacaine.82 There are fewer pediatric trials available in literature. Because of the common use of bupivacaine in children and its low incidence of complications, levobupivacaine is not used abundantly in general pediatric anesthesiology practice. It is currently not available for use in the United States, although it is widely used in other parts of the world.
Toxicity
Levobupivacaine, in the animal model, has been shown to have less cardiac toxicity with lower degree of myocardial depression than bupivacaine.83
Esters
Ester local anesthetics are metabolized by plasma cholinesterases.84 As a result, in populations with lower pseudocholinesterases as in neonates, we see an increase in the duration of local anesthetic activity. This includes infants and neonates particularly. The duration of action of the drug is limited; hence, a continuous infusion of chloroprocaine is recommended.
Toxicity
Toxicity is based on the absence of pseudocholinesterase in the neonate.
Dosing
After a bolus dose of 1 mL/kg, a continuous infusion of chloroprocaine at 0.3 mL/kg of a 3% 2-chloroprocaine is recommended to achieve a level of T4 to T2.85 This will be effective in producing complete surgical anesthesia for neonates undergoing hernia repair. Although the drug is not commonly used in pediatric practice, the advantage of its use is the capacity to provide complete motor block that is not prolonged.
Management of Local Anesthetic Toxicity
Lipid emulsion therapy has been demonstrated to reverse the effects of local anesthetic toxicity in experimental animal models.86 Its effect on human models was parlayed in a case report where lipid was used as a last resort to reverse the effects of bupivacaine following a regional anesthesia technique.87 Lipid should be readily available if local anesthesia is being used in infants. More recently an infant receiving a caudal block which resulted in cardiac toxicity was treated successfully with lipid rescue.88
Topical Anesthesia
Several local anesthetic preparations are now available for topical use. The most common local anesthetic preparations for topical use include lidocaine, tetracaine, benzocaine, and prilocaine. When these are applied to skin they produce effective but relatively short duration of analgesia. A topical anesthetic formulation EMLA (eutectic mixture of local anesthetic) is a mixture of lidocaine 2.5% and prilocaine 2.5%89 and is used extensively for topical anesthesia in neonates, particularly for circumcision and venipunctures. The preparation has to be applied under an occlusive bandage for 45 to 60 minutes to obtain effective cutaneous analgesia. Although the incidence of methemoglobinemia from prilocaine is not very common in neonates, caution should be exercised when applying large doses of EMLA and caution should be exercised while applying large doses for procedures.90
Newer topical anesthetic solutions are now available that may offer a faster rate of onset. LMX-4, a 4% liposomal lidocaine solution can be used as topical anesthesia. There is no need for an occlusive dressing when LMX-4 is used, and it has the same efficacy as EMLA.91,92 Liposome-encapsulated lidocaine or tetracaine has been shown to remain in the epidermis after topical application, affording a fast and lasting anesthetic effect.
Anesthetic Management of the Neonate
Effective evaluation, preparation, and anesthetic management of the neonate depend on appropriate knowledge, clinical skills, and vigilance by the anesthesiologist. For safe and effective care, the anesthesiologist must take extraordinary care to understand the current status of the patient, the nature of the planned surgery, and the potential need for stabilization and preparation before surgery. After ensuring that the patient has been adequately prepared, the anesthesiologist needs to develop a detailed plan that encompasses the issues of anesthetic equipment and monitoring, airway management, drug choice, fluid management, temperature control, anticipated surgical needs, pain management, and postoperative care.
Studies have shown that morbidity and mortality related to anesthesia is higher in infants, especially neonates, compared with infants, older children, and adults.93,94,95,96 There are probably
several causes for this higher complication rate, including the emergent nature of most surgical procedures that are performed at this age, the physiologic instability of the neonate, the relative lack of experience most clinicians have with patients in this age range, and the technical challenges of monitoring and treating a very small patient. Because of the specialized nature of neonatal surgery and care, it is important that each institution that provides care to these patients have the resources of equipment, critical care facilities, nursing, laboratory, blood bank, and social work necessary to meet the needs of these patients and their families, as well as systems in place to guarantee a robust quality assurance emphasis on the provision of care. Both the American Academy of Pediatrics and the American Society of Anesthesiologists have provided guidance to many of the systems issues that should be addressed in institutions caring for these patients.97 Physicians who agree to participate in this care need to have the preparation and ongoing experience needed to provide a consistent, high level of care.
several causes for this higher complication rate, including the emergent nature of most surgical procedures that are performed at this age, the physiologic instability of the neonate, the relative lack of experience most clinicians have with patients in this age range, and the technical challenges of monitoring and treating a very small patient. Because of the specialized nature of neonatal surgery and care, it is important that each institution that provides care to these patients have the resources of equipment, critical care facilities, nursing, laboratory, blood bank, and social work necessary to meet the needs of these patients and their families, as well as systems in place to guarantee a robust quality assurance emphasis on the provision of care. Both the American Academy of Pediatrics and the American Society of Anesthesiologists have provided guidance to many of the systems issues that should be addressed in institutions caring for these patients.97 Physicians who agree to participate in this care need to have the preparation and ongoing experience needed to provide a consistent, high level of care.
In the distant past, concerns about physiologic instability and other challenges of caring for neonates led some practitioners to use minimal or no anesthesia for both minor and major procedures.98,99,100 It is now widely recognized that neonates have stress responses similar to those of older patients, and the lack of adequate anesthetic care is as inhumane in the neonate as it is in the older child or adult.101 Consequently, the same attention to adequate analgesia and anesthesia needs to be paid to the neonate as to other patients.
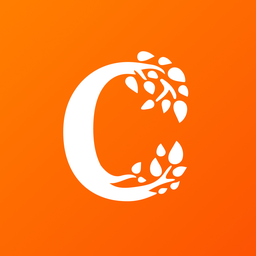
Full access? Get Clinical Tree
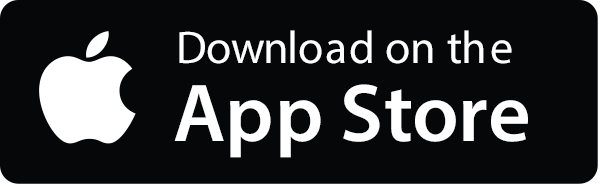
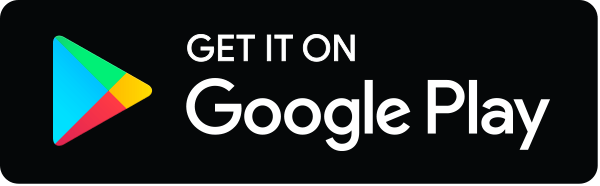