Chapter Outline
Automated Determination of Respiratory Rate
Thoracic Impedance and Inductance
Electrocardiograph-Based Respiratory Monitoring
Breathing Circuit Low-Pressure Alarms
Sensor Position Within the Breathing Circuit
Airway Versus Alveolar Pressures
Ventilation Mode Effects on Airway Pressures
Flow-Volume and Pressure-Volume Loops
Compliance and Resistance Optimization
Loops Related to Equipment Issues
CAPNOGRAPHY AND VOLUMETRIC CAPNOGRAPHY
Overview
Until the early twentieth century, animal life was defined by the presence of spontaneous ventilation. Absence of breathing implied death or impending death, although experiments with resuscitation and artificial ventilation had occurred since the eighteenth century. The defining characteristics of spontaneous ventilation , depth and frequency, were early indicators of anesthetic action, with profound depression of respiration indicating the deepest plane of ether narcosis.
The most fundamental measure of ventilation is frequency, measured in units of inverse time, usually min −1 . Although physiology pundits will remind us that respiration is a cellular phenomenon, the term respiratory rate is commonly accepted to denote the frequency of ventilation, either spontaneous or controlled.
Respiratory Rate
Respiratory rate is still commonly assessed by direct observation, either by counting the spontaneous breaths observed in a full minute or by doubling the count in 30 seconds. In the nearly well patient, the frequency at rest is relatively predictable (~14 to 18 min −1 ), whereas in the critically ill or perioperative patient, it is more variable. In reference to hospital patients in the 1950s, one commentator noted that almost all patients had recorded “respiratory rates” of between 18 and 22 min −1 , irrespective of their actual value; this led him to conclude that the routine recording of respiration was not only useless but wasted millions of hours of health care time annually. On the other hand, a more astute observer commented that respiratory rate “is a sensitive clinical parameter in a multitude of pulmonary diseases, especially in the critical care setting.”
Although tachypnea has many underlying causes, one common perioperative cause is pain. It is unusual for severe, acute pain not to be accompanied by hyperventilation as a part of a clinical picture of systemic stimulus and sympathetic nervous system activation. Conversely, patients who have recently received opioid analgesics may exhibit bradypnea as one sign of a relative overdose. Thus the frequency of spontaneous ventilation may be a crude but valuable tool in preserving the comfort and safety of perioperative patients in that it may express the balance between acute pain and analgesic effects. In a spontaneously breathing patient, the titration of opioid analgesics to effect is much more difficult without access to this simple, real-time variable.
Automated Determination of Respiratory Rate
Determination of respiratory rate by direct observation is difficult because of interobserver variability, length of time needed for accurate readings (up to 1 minute), and the confounding effects of upper airway obstruction that produce chest wall motion and apparent breaths in the absence of gas exchange. The reliable automation of this data gathering is valuable, not only to perioperative caregivers—especially those who perform regional or sedation techniques—but also to nonanesthesiology personnel responsible for patient sedation in more remote or ambulatory locations.
Instrumentation to measure respiration may be direct, as in airflow sensors—pinwheels, gas sampling, or thermocouples—or indirect, imputing air flow from changes in thoracic dimension or breath sounds. Indirect measurements of chest volume may be via strain gauges, impedance measurements, or electrocardiograph (ECG) analysis. In a fully equipped operating suite, the most convenient method of measurement is with a capnograph. In the tracheally intubated, anesthetized patient and in the awake or sedated patient, exhaled gas flow may be sampled using a number of devices. In the case of the spontaneously breathing patient, a nasal cannula may be configured to both sample exhaled gas and simultaneously deliver supplemental oxygen.
Thoracic Impedance and Inductance
An early attempt to automate respiratory rate measurement in the 1960s was based on the theory that the electrical properties of the thorax changed during the respiratory cycle. The resistance to the flow of alternating current, known as impedance, was found to change with the inflation and deflation of the lungs; it is increased with inhalation. Because of this, this impedance value could be continuously measured; when it exceeded a set threshold, the occurrence of a breath was recorded by a monitor. Efforts were made to correlate changes in impedance with tidal volume, but this was less successful in awake patients and in those with respiratory disease. Significantly, this technique does not measure actual air entry but only measures the changes in the shape of the thoracic cage. Accordingly, it could be fooled by respiratory effort in the presence of upper airway obstruction. In anesthetized and ventilated surgical patients, however, the technique was much more valuable, producing accurate respiratory rate measurements and high correlation of tidal volumes (r = 0.89) with Wright’s respirometer. In addition, the technique was somewhat sensitive to both airway occlusion and circuit disconnects.
Work has been done to determine the optimal placement of electrode pairs for maximum sensitivity. As expected, maximal separation of electrode pairs provides the most sensitive signal. Interference between respiratory monitors that use thoracic impedance and implanted respiratory rate–sensitive pacemakers has been reported, producing unwanted heart rate changes. The only solution was to disable the respiratory rate monitoring function in the physiologic monitor.
Inductive plethysmography is superficially similar to impedance plethysmography in that electrically active sensors detect chest wall movement. However, the inductive technique uses direct-current sensors in the form of coils of wire around the chest and/or abdomen. The changes in self-inductance (stretch) of these sensors are electrically transmitted and analyzed. These signals can reflect respiratory rate and, after calibration, tidal volume. In a study of infants at risk for apnea, Brouillette and colleagues found that inductive plethysmography was superior to impedance-based measurement in that it recorded fewer cardiac artifacts and was better able to discern upper airway obstruction.
Electrocardiograph-Based Respiratory Monitoring
After the use of continuous ECG monitoring became widespread, attempts were made to use ECG-derived data to record respiratory rate. The ECG may be used through the analysis of changes in both the amplitude of the ECG signal and the changes in the axis of the QRS complex. Amplitude changes in the ECG are caused by the change in overall electrical resistance because of the increased proportion of air, rather than tissue, within the chest cavity during inspiration. This is reflected in a decrease in ECG signal voltage, and thus QRS wave size, that is amenable to analysis.
Inflation of the lungs also produces a change in the axis of the heart, as reflected in the multilead ECG. Using two limb leads, measurements are made of the area under a normal QRS complex. By using these areas as amplitudes of known direction, a vector can be derived as the sum of the two values. These measurements are routinely made by most arrhythmia detection software in current use.
Other Techniques
Acoustic techniques actually “listen” for the sound of airflow at the face, pretracheal neck, or chest. One method uses the sound of air impinging on open-ended tubes positioned near the nostrils and mouth. Although lightweight and immune to upper airway obstruction artifact, this method may suffer from interference from ambient noise. Also, it is a pure respiratory frequency monitor, without any other measure of ventilatory adequacy. A variation of this approach uses a pyroelectric polymer mounted inside a face mask that senses the temperature increase from exhaled air. This signal is electrically amplified and displayed. Inexpensive and relatively simple, it can be incorporated into the construction of a face mask or nasal cannula.
A sophisticated, noninvasive sensor was described by Zhu and colleagues in 2006. A head pillow containing fluid-filled tubes is connected to two sensitive pressure transducers. The pressure signal is processed with a derivation of an algorithm known as wavelet transformation and is filtered to produce both pulse and respiratory signals. The technique is, as expected, less effective in the presence of motion artifact and poor head positioning. However, in 13 healthy subjects, both the sensitivity and the positive predictive value of the sensor were above 95% for respiration, compared with simultaneous nasal thermistor recordings. The data were even more favorable with respect to detection of pulse rate. In combination with oximetry, this technique will likely prove valuable both in ambulatory monitoring and in the diagnosis of sleep disorders.
The Rainbow Acoustic Monitoring system (Masimo, Irvine, CA) noninvasively and continuously measures respiration rate using an innovative adhesive sensor with an integrated acoustic transducer that is easily and comfortably applied to the patient’s neck. Using acoustic analysis, the respiratory signal is separated and processed to continuously display respiration rate. Box 9-1 summarizes the various methods for automated detection and recording of respiratory rate.
Direct
Airflow Sensors
Pneumotachograph
Thermistor
Differential pressure (Bernoulli)
CO 2 waveform
Acoustics
Mouth/nose
Precordial
Pretracheal
Neck (Masimo)
Indirect
Chest Wall Motion
Strain gauge
Impedance change
Cardiac Derived
Electrocardiograph voltage variation
Cardiac axis change
RR interval change
Blood pressure variability
Other Body Motion
Pillow sensor
Airway Pressures
Principles Overview
Pressure is defined as the force per unit area exerted on a surface by an external substance, usually a gas or liquid in medical applications. A molecule of a gas can be envisioned as confined within a cube. This molecule of gas transfers its momentum to a vessel wall during a collision equal to 2 × mv, where m is mass and v is mean velocity. After bouncing off the opposite wall, the molecule then hits the first wall again after a time equal to 2 × d/v, where d is the distance between walls, exerting a force of mv 2 /d. With N molecules, one third will hit each pair of sides of the box, yielding a force ( F ) on each wall:
F = ( Nmv 2 / d ) ÷ 3
P = ( Nmv 2 / d 3 ) ÷ 3
F = ( Nmv 2 / V ) ÷ 3
This gives the useful fact that, at constant temperature, pressure is inversely proportional to volume (Boyle’s law) and directly proportional to the square of molecular velocity.
Determination of atmospheric pressure was the first practical application of pressure gauges. Initially, the force exerted by the weight of the atmosphere was balanced against a column of fluid, either water or mercury. This is an example of an absolute pressure gauge, indicating approximately 30 inches (or 760 mm) of mercury, 101 kPa, or 1.01 bars of pressure at sea level. In contrast, relative or “gauge” pressure indicators read zero at atmospheric pressure and indicate only additional pressure sensed.
Sensors
The history of airway pressure measurement parallels the development of sensitive gauges and meters for atmospheric pressure. Beginning with fluid columns, devices for the estimation of gas pressure rapidly evolved into mechanical linkages translating physical motions—of an evacuated capsule, for example—into displacements of an indicating needle. The Bourdon gauge ( Fig. 9-1 ) uses this principle of change in pressure, translated into motion of an evacuated compartment or tube, and is still a robust and inexpensive mechanism. This mechanical gauge, and others similar to it, require no power, are always on, and have high reliability. Although they do not communicate with data systems or alarms, they are useful backups for modern electronic systems.

Newer electronic sensors use the principle of piezoelectricity, the property of certain crystals, usually quartz, to produce a small electric current when compressed. This output may be calibrated and amplified into a usable signal. A metal or semiconductor whose resistive properties change with strain, a so-called piezoresistive effect, also may be used to sense pressure. This additional technologic development has facilitated the evolution and miniaturization of pressure sensors that may be adapted to almost any application. In general, these sensors do require periodic calibration against atmospheric pressure (zero) and occasionally require one other known data point (span) to ensure linearity across their operational range. They are small, lightweight, and generally much more sensitive to small changes.
Applications
Airway pressure is a key component in measuring ventilation. Detecting ventilator-related events (VREs) helps anesthesia providers identify and avoid a majority of serious anesthesia-related events. Disconnection of the circuit without occlusion is a significant anesthesia-related event that may lead to adverse patient outcomes.
Pressure sensors have been used to detect other mechanical problems, including tracheal tube disconnection with occlusion, kinking of the inspiratory limb, fresh gas hose kink or disconnection, circuit leaks, high and low scavenging system pressure, continued high circuit pressure, and kinking in the circuit pressure-sensing hose. However, the ability to detect these conditions, especially with older monitors, is not as reliable.
Before the introduction of these sensors, previous techniques included lung auscultation, measures of chest wall movement, use of a precordial or esophageal stethoscope, and measurement of physiologic variables such as pulse, blood pressure, respiration, and skin color. These other monitoring methods, absent end-tidal carbon dioxide and pulse oximetry, often resulted in delayed or failed detection of events.
Ventilation failure events can occur because positive pressure does not always equal tidal volume delivered. Sensors in different locations along the breathing circuit, especially with pressure-based ventilator modes, can give disparate pressure measurements and are thus susceptible to both false-negative and false-positive readings.
Breathing Circuit Low-Pressure Alarms
At a minimum, a low-pressure alarm with an audible alert must be placed in the patient breathing circuit in accordance with the current American Society of Anesthesiologists (ASA) Standards for Basic Anesthetic Monitoring. Pressure-sensitive alarms monitor airway or breathing circuit pressure through a side port and compare it with a preset low-pressure alarm limit. The primary purpose of this alarm is the prompt identification of breathing circuit disconnection. This class of malfunction has been disproportionately associated with adverse outcomes in the intraoperative period and may be almost completely avoided when this relatively simple monitor is used. Although low-pressure alarms are primarily intended to warn of a breathing circuit disconnection when a positive-pressure ventilator is used, it is important to realize that they do not detect some partial disconnections and will likely not detect misconnections or obstructions. However, because 70% of all disconnections occur at the Y-piece, it is an excellent addition to the other sensors used to monitor ventilation.
Sensor Position Within the Breathing Circuit
The location of airway pressure sensors is important to both detect circuit disconnects and accurately reflect distal airway pressures. Sampling location, kinetic energy transfer, and Bernoulli effects all influence final measured pressure. Circuit pressure should be measured at an appropriate site for the particular need and at a right angle to axial flow. The ideal location is at or near the patient Y-piece, but secretions and condensation may cause sensor malfunction. If it is not close enough to the Y-piece, an undetected disconnection (false-negative) may occur as a result of circuit resistance between the measurement site and the distal, now open, end. This will be compounded if a descending bellows ventilator is used, or if the disconnection site is embedded in sheets, blankets, or some other obstructing material.
A sensor inside the circuit, upstream of the inspiratory valve, eliminates any risk of contamination but becomes insensitive under certain conditions. The combination of an inspiratory limb low-pressure monitor with an in-line humidifier, capnometer cuvette, angled tracheal tube connector, or corrugated catheter mount can create backpressure with medium to high flows and may prevent the alarm from being activated. Increased backpressure has also been seen in the Bain (or C-Pram) breathing circuit as a result of circuit tapering and extension of the fresh gas outlet to near the connector at the patient. Lower flows can also prevent the alarm from being activated if moisture builds in the in-line humidifier.
One proposed solution is to place the low-pressure alarm on the expiratory limb to avoid backpressure-induced false-negative events. However, expiratory limb pressure sensors are not without problems. Apnea volume alarms have also been sounded, without the pressure alarm sounding, as a result of loose retaining rings in the expiratory limb not detected during machine leak testing. Interestingly, reverse-flow and minute volume low alarms may trigger without a low-pressure alert. In older anesthesia delivery systems without fresh gas compensation, expiratory valve anemometers routinely overestimated tidal volumes because of high flows of fresh gas passing through the circuit during the expiratory phase.
Pressure sensors within the ventilator obviously are insensitive in machines with a manual/automatic selector switch in the manual position. The placement of pressure sensors in both the circuit and ventilator may provide a solution, but this has been implemented only in the Medical Anesthesia Delivery Unit (ADU) anesthesia machine (GE Healthcare, Waukesha, WI). Recently, ventilation pressure sensors have been placed in both the inspiratory and expiratory circuit limbs. The inspiratory limb sensor provides feedback during volume-controlled ventilation. Stress fractures in these sensors may trigger alarms without directing the provider to the site of the fault. The small fracture size prevents detection through low- and high-pressure leak tests. Options include detection through visual inspection, using alcohol-wet hands to detect a small gas leak, or using a second reservoir bag as a test lung to verify sensor integrity.
To improve the rate of detection of true-positive events, the low-pressure limit should be set just below the normal peak airway pressure, and subsequent machine testing should establish that a disconnection would cause the alarm to sound. The disconnection should be performed at the distal (patient) end to ensure that backpressure, flows, and monitor limits are not such that the alarm would fail to sound in the event of a disconnection.
The clinical response to a low-pressure alarm should be rapid and organized. The anesthesiologist must systematically evaluate the gas flow to ensure it is adequate, the breathing circuit to ensure it is not disconnected or leaking, and the ventilator, which may not be driving the bellows or may have a setting error. Working outward from the adjustable pressure-limiting (APL) valve toward the patient helps the anesthesiologist rapidly identify and correct the issue.
Airway Versus Alveolar Pressures
With controlled ventilation, a transpulmonary inflation gradient is provided by positive airway pressure. Generated by a ventilator, this positive pressure gradient may be constant or may vary during the inspiratory phase. The instantaneous pressure during the ventilation cycle is best measured at the distal end of the ventilator circuit, near the connection to the patient. Division of the airway pressure-time product (area under the curve) by total cycle time yields mean airway pressure. In most cases,this average pressure approximates mean alveolar pressure. Mean airway pressure also correlates with alveolar ventilation, arterial oxygenation, hemodynamic performance (venous return), and risk of barotrauma.
Alveolar pressure is clinically of interest, but direct measurement generally is not practical. The measurement requires measurement of airway pressure at the distal portion of the endotracheal tube, as close as possible to the alveoli, whereas clinical airway pressure measurements commonly are at the proximal portion of the endotracheal tube. Measured airway and actual alveolar pressures differ because of proximal dissipation of the frictional inspiratory airway pressure component and the expiratory alveolar pressure resistive contributions. Positive end-expiratory pressure (PEEP) adds to the external circuit pressure throughout the cycle, proportionally increasing the mean airway pressure.
Chest wall and lung compliance, secretions, partial occlusions, and tracheal tube resistance may also affect peak airway pressures. Plateau pressures are measured during an inspiratory pause (no flow) and are affected only by secretions, partial occlusions, and tracheal tube resistance.
Ventilation Mode Effects on Airway Pressures
Volume control will deliver a set tidal volume, and pressure will increase until that volume is reached. In low lung compliance conditions, such as acute respiratory distress syndrome (ARDS), high pressures can be administered in attempts to deliver a set volume.
Pressure control will deliver a set pressure, and the tidal volume will depend on airway resistance and lung and chest wall compliance. Pressure control may be better tolerated in some patients, improving both ventilation and oxygenation. However, if lung compliance decreases, and the same pressure is applied, the tidal volume will decrease. Appropriate volume alarm settings will signal low volumes and avoid undetected hypoventilation.
Pressure support mode delivers a positive pressure that is rapidly achieved and maintained throughout inspiration whenever the patient makes an inspiratory effort. The volume delivered depends on the pressure setting, inspiratory time and effort, and airway resistance and compliance.
Pressure Alarms
Subatmospheric Pressure Alarms
Subatmospheric alarms measure and alert the clinician to a subatmospheric (negative) circuit pressure and the potential for reverse flow of gas. Negative pressures can rapidly cause pulmonary edema, atelectasis, and hypoxia. Commonly, the low pressures result from active (suction) scavenging system malfunctions or patient inspiratory efforts against a circuit that is either blocked or that has inadequate fresh gas flow. Other events detected include suction applied to a gastric tube that has been passed into the trachea alongside the tracheal tube and moisture accumulation in the carbon dioxide absorber that decreases gas flow to the patient.
High-Pressure Alarms
High-pressure alarms are now user adjustable or even automated, but in older monitors, this was not always the case. High respiratory rate, low inspiratory/expiratory ratios, low tidal volume, low flow of inspiration and fresh gas, and high tubing compliance may result in a failure to trigger the alarm. Older machines may generate excessive pressure and put the patient at risk without exceeding their preset level, usually near 40 cm H 2 O. Positive inspiratory pressure (PIP) measurement and adjustable alarms are especially valuable in the pediatric population. Endobronchial intubation can be identified rapidly using pressure monitoring before the onset of hypoxia or hypercapnea.
Continuing Pressure Alarms
A continuing pressure alarm is triggered when circuit pressure exceeds 10 cm H 2 O for more than 15 seconds, and it alerts the anesthesiologist to more gradual increases in pressure, such as those that result from a ventilator pressure relief valve malfunction (a valve stuck closed) or a scavenging system occlusion. In these situations fresh gas continues to enter the breathing system from the machine flowmeters but is unable to leave. Rate of rise of pressure therefore depends on the fresh gas flow rate.
Modern anesthesia machines now have multiple pressure sensors, as described. In addition to the electronic sensors in the ventilator and the circuit, an analog gauge usually is positioned on the carbon dioxide absorber to serve as a quick reference and as a backup in case of electrical failure.
Volume Measurement
Principles and Requirements
In the management of controlled ventilation, it is important to monitor minute ventilation—the volume of gas delivered to the airway in 1 minute—as a measure of ventilatory “adequacy.” This measure is best obtained by measuring tidal (breath) volume and then multiplying by respiratory frequency. Because arterial partial pressure of carbon dioxide is inversely proportional to alveolar minute ventilation, this measurement is important both for initial ventilator setup and subsequent adjustments. Measurements may be made intermittently with manual methods; more commonly, they are made with a device integrated into a ventilator circuit and electronically monitored. This integration may include the derivation and display of minute ventilation, flow-volume loops, and determinations of pulmonary compliance.
Dead Space
Because not all tidal ventilation delivered reaches the alveoli, effective gas exchange occurs only in a fractional part of the tidal volume. The remaining gas occupies the trachea, bronchi, and bronchioles and is referred to as anatomic dead space . This volume is relatively fixed in each patient; thus, as tidal volume decreases, it occupies a larger fraction of each breath. At a tidal volume roughly equal to the anatomic dead space, effective alveolar ventilation ceases, with the same gas being transported distally from bronchi to alveoli that was most recently expelled. Also, the distal breathing circuit and patient airway contribute additional mechanical or apparatus dead space to the system ( Fig. 9-2 ).

Measurement of dead space is important to minimize its contribution to total minute ventilation and clearly calculate the relationship between alveolar minute ventilation and arterial carbon dioxide tension. A combination of precise volume measurement and capnography may be used to calculate anatomic dead space using Fowler’s method. Initially described using nitrogen as the reference gas, the expired volume was measured from the beginning of exhalation until the onset of the “plateau” of alveolar gas.
Mechanical Devices
The simplest device for the measurement of gas volume is based on a rotating vane or propeller calibrated against a specific density of gas. The total rotation of the attached shaft correlates with the volume of gas (air) that has passed by. The basic principle is that a force is transmitted to the vanes by the impact of gas molecules, and this force is converted to a rotational (angular) momentum and spins the pinwheel. Optical or mechanical transducers count the rotations and convert the value to an equivalent volume. Critical variables are the gas density—which depends on gas composition, humidity, and altitude—and temperature. At very low flows or volumes, the device may be less accurate as a result of the finite mass and inertia of the vanes.
Wright’s Respirometer
Introduced in 1955, this vane anemometer uses a low-mass rotating vane that responds to the force of flowing gas by rotating in proportion to flow. It records tidal volume, which is proportional to total rotations, and it can sum multiple breaths to indicate minute volumes. Manufactured by several companies in both mechanical and optically encoded forms, the overall shortcoming of this device is volume inaccuracy at low flows. Low flow causes the turbine to accelerate more slowly because of inertial effects, so the accuracy is lower. It is considered a very safe monitor, however, because it understates inspired tidal volumes at low volumes and continuous low flows as a result of gas slippage past the vanes, although expired volumes continue to be reliable. Pulsatile flows will cause an overread of tidal volume because of higher peak flow rates and “coasting” of the vane between breaths. The Ohmeda 5400 Volume Monitor (GE Healthcare), an optically encoded turbine, is the exception in this class. It overreads with low flows and underreads with high flows.
The Wright respirometer (Cardinal Health, Dublin, OH) design is not ideal for low-flow anesthesia because it requires a minimum flow of approximately 2 L/min. The monitor is also not appropriate for spontaneously breathing pediatric patients, whose tidal volumes are insufficient to rotate the vane. If pediatric patients are artificially ventilated, the device may be more useful; this is because with expiratory valve opening, the initial flow rates should be sufficient to overcome inertia and rotate the vane. Hatch studied a modified turbine design that is accurate at volumes of 15 to 200 mL; it also is electronically encoded and incorporates an integral apnea alarm.
Several manufacturers have used this technology to measure expiratory volumes at the proximal end of the expiratory limb of a breathing circuit. Head-to-head comparisons of the various devices showed reasonable reliability of this class of tidal volume monitor above minute ventilations of 5 L/min using air, nitrous oxide/oxygen, or humidified air. As minute ventilation decreases, the measured tidal volume will be less than the actual volume delivered; however, the accuracy of the device depends on the transducer used by the manufacturer ( Fig. 9-3 ).

A sealed mechanical volumeter ( Fig. 9-4 ) was used by Dräger Medical (Telford, PA) in some older delivery systems to measure tidal volume and minute ventilation. This device consists of a pair of rotating elements in the gas flow path, configured much like a revolving door at the entrance to a building. A fixed volume of gas is passed across the volumeter with each quarter rotation of the dumbbell-shaped elements. A seal is formed between the polystyrene rotating elements and the interior wall of the tube, and a sealed volumeter provides substantially more resistance to flow than does the vane anemometer. The accuracy of this volumeter is affected by gas density and by the inertia of the rotating elements; however, it is not influenced substantially by the flow pattern of the gas. The number of fixed volumes of gas transferred from inlet to outlet is mechanically measured.

The Dräger Spiromed is an electronic version of the mechanical spirometer. Gas flow through the device determines the rate of rotation of the rotors, which is measured by an electromagnetic sensing system. The Spiromed is direction sensitive and can alert to reversal of gas flow in the circle system. The accuracy of the Spiromed for tidal volumes is ±40 mL and ±100 mL for minute ventilation, or 10% of the reading.
Measurement of Gas Flows
The flow of any fluid material, liquid or gas, may be described by a set of equations that embody the concepts of smoothness, continuity, and conservation of mass. This description allows for properties such as density, viscosity, compressibility, mass, and volume. As fluids flow, they interact with their surroundings—that is, other fluid or container walls—in predictable ways. An important concept is the distinction between laminar (smooth) and turbulent (chaotic) fluid flow. This is an interesting exception to the normally smooth, continuous nature of fluid transport.
Turbulent flow is characterized by the formation of eddies or vortices on an increasingly small scale. The transition from laminar to turbulent flow is catalyzed by increasing drag on the fluid by friction from interaction with boundary layers, walls, or orifices in the fluid path. It is a chaotic process that depends on a complex combination of fluid density, viscosity, and the size and shape of the obstruction to flow.
Principles
The behavior of fluids and gases depends on whether their flow is laminar (smooth) or turbulent. Flow through a tube with a length greater than the diameter usually is laminar; however, at a critical velocity (V C ), a transition to turbulent flow occurs. This is shown in the following equation, in which r is the radius of the tube, ⍴ is the density, η is the viscosity of the gas, and k is the critical Reynolds number, which is different for each gas:
V c = k η / ρ r
This transition to turbulent flow greatly increases the resistance to flow and may be seen in many common scenarios, such as a kink occurring in an otherwise smooth tracheal tube. For flow through an orifice, in which length is less than the radius, turbulent flow is a given. Measured in units of length cubed per unit of time, volume flow may be calculated by detecting a velocity change across a fixed cross-sectional area. Bernoulli’s equation makes this possible by measuring a pressure change across this transition:
P + 1 2 ρ V 2 + ρ gh = Constant
P 1 − P 2 = 1 2 ρ ( V 2 2 − V 1 2 )
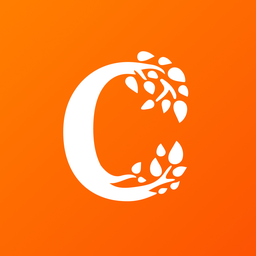
Full access? Get Clinical Tree
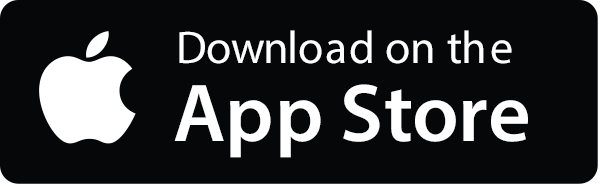
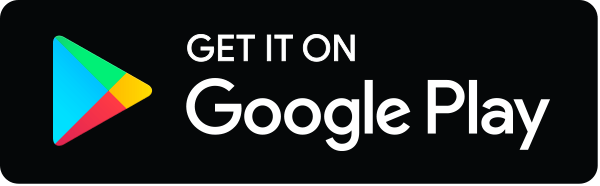