Chapter 14 Monitoring oxygenation
THE ROLES OF OXYGEN IN AEROBIC ORGANISMS
Oxygen has several vital physiological roles:
TISSUE HYPOXIA
As tissue PO2 falls, the biosynthetic and biodegradation systems are the first to succumb. Cell signalling by reactive oxygen species, released at mitochondrial complex III, activates hypoxia-inducible factor-1, a transcription factor upregulating genes important in hypoxic cell survival.3 Oxidative phosphorylation starts to fail at an intracellular PO2 of 0.1–1 mmHg, equivalent to an extracellular PO2 of around 5 mmHg. At this point oxygen-limited cytochrome turnover causes progressive ATP depletion, a process which has been termed ‘dysoxia’.4 Stopgap production continues by anaerobic glycolysis, but without correction of dysoxia there is progressive lactic acidosis and eventual cell death by apoptosis and necrosis. On reoxygenation, further release of reactive oxygen species causes oxidant stress, often overshadowing the hypoxic insult.2
INSPIRED GAS
Monitoring the fraction of inspired oxygen (FiO2) is necessary to prevent both hypoxaemia and the adverse effects of excess oxygen. The inspired oxygen tension (PiO2) of humidified gas is determined by the FiO2, the barometric pressure (BP) and the saturated vapour pressure of water (47 mmHg).
TRANSFER OF INSPIRED GAS TO ALVEOLI
Communication between oxygen delivery system and pulmonary alveoli is open if:
ALVEOLAR GAS
DISTRIBUTION OF ALVEOLAR VENTILATION
Clinicians routinely track chest movement, auscultate air entry and examine plain chest radiographs. Although computed tomography scanning can reveal occult overdistension,7 it has logistical disadvantages and is a significant radiation hazard. Electrical impedance tomography is under development as an alternative, and shows promise.8 Simple and non-invasive, it tracks lung volume changes in real time, with potential to optimise alveolar ventilation distribution while limiting overdistension.9
MATCHING OF VENTILATION AND PERFUSION
For efficient gas exchange, the majority of lung units must have well-matched alveolar ventilation and perfusion (V/Q ratios close to 1). Even in health there is a spread to lower and higher V/Q ratios, all clustered around unity. When lungs are diseased, V/Q scatter is much greater, with variable representation across the full spectrum from zero to infinity. Such complexity resists simple bedside quantification. The best method is the multiple inert gas technique (MIGET). MIGET generates a lung model with 50 compartments spanning the full range of V/Q ratios, by measuring the retention and elimination of six inert gases of varying solubility.10,11 Because MIGET is impractical at the bedside, simpler non-invasive modelling of shunt and V/Q mismatch is under evaluation, using FiO2 as a forcing function while tracking haemoglobin oxygen saturation.12 Predictive capacity13 and ease of application14 are encouraging.
THE IDEAL ALVEOLUS AND THE THREE-COMPARTMENT LUNG MODEL
The alveolar PO2 in the ideal compartment (PAO2) is calculated from the alveolar gas:
where R is the respiratory exchange ratio, either measured by indirect calorimetry or assumed to be 0.8. PiO2 is calculated as in Equation 14.1. PaCO2 is arterial PCO2.
Most clinicians use the following approximation:
TRANSFER FROM ALVEOLI TO ARTERIAL BLOOD (PULMONARY OXYGEN TRANSFER)
The MIGET technique has identified V/Q mismatch and intrapulmonary right-to-left shunt as the two main causes of reduced pulmonary oxygen transfer in critical illness.15 Intrapulmonary shunt predominates in the acute respiratory distress syndrome (ARDS), in lobar pneumonia and after cardiopulmonary bypass, whereas V/Q mismatch without shunt is more prominent in chronic lung disease.16
BEDSIDE INDICES OF PULMONARY OXYGEN TRANSFER
These are either tension-based or content-based.
TENSION-BASED INDICES
A-a gradient
The A-a gradient is calculated as PAO2 – PaO2, where PAO2 is the ‘ideal’ compartment alveolar PO2 determined from the alveolar gas equation (Equation 14.2). Hypoxaemia can then be classified under two headings:
Raised A-a gradient
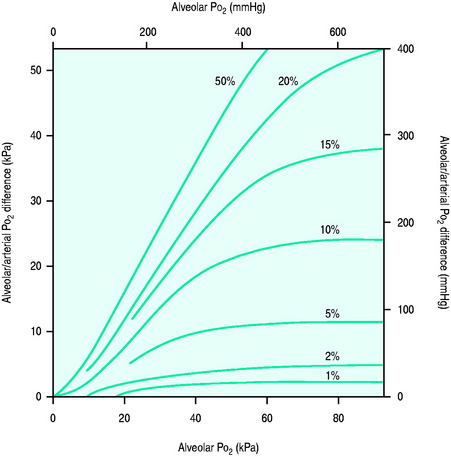
Figure 14.1 Effect of varying FiO2 (PAO2) on A-a gradient with different degrees of intrapulmonary shunt.
(Reproduced from Nunn JF. Oxygen. In: Nunn JF (ed.) Applied Respiratory Physiology, 4th edn. Oxford, UK: Butterworth-Heinemann; 1993: 264, with permission.)
Pao2/Fio2 ratio
The PaO2/FiO2 ratio is used to define acute lung injury and ARDS,18 and is an input variable in the Simplified Acute Physiology Score (SAPS II)19 and lung injury scoring systems.20 At sea level its normal value is ≥ 500 mmHg. In acute lung injury, PaO2/FiO2 < 300 mmHg, whilst in ARDS the ratio is < 200 mmHg.
Its only advantage is simplicity. There are several major disadvantages:
CONTENT-BASED INDICES
Venous admixture (Qs/Qt)
Cc’O2, CaO2 and CvO2 are the oxygen contents of pulmonary end-capillary, arterial and mixed venous blood respectively. CaO2 and CvO2 are calculated using data from arterial and mixed venous blood gas analysis and CO oximetry (see Table 14.4, below). Cc’O2 is derived differently, since pulmonary end-capillary blood cannot be sampled. is assumed to equal PAO2 as derived from the alveolar gas equation (Equation 14.2).
(normally close to 1) can then be computed from an algorithm for the HbO2 dissociation curve.22
Disadvantages of venous admixture
When determined at FiO2 = 1, venous admixture is an accurate measure of right-to-left shunt. However, exposure to 100% oxygen causes absorption atelectasis of unstable low V/Q units and increases intrapulmonary shunt.
Estimated shunt fraction
If a fixed CaO2 – CvO2 can be assigned, no PA catheter is necessary. However, in critical illness CaO2 – CvO2 can range from 1.3 to 7.4 ml/dl. The inaccuracy from assigning a fixed value is such that even the direction of change of estimated shunt can be wrong.23
ARTERIAL BLOOD
Indices of arterial oxygenation are PaO2 and SaO2. They are linked by the HbO2 dissociation curve (Figure 14.4).
< div class='tao-gold-member'>
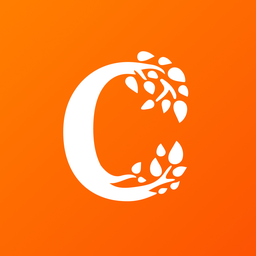
Full access? Get Clinical Tree
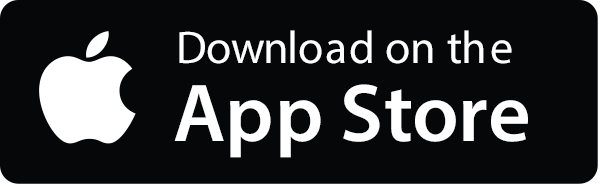
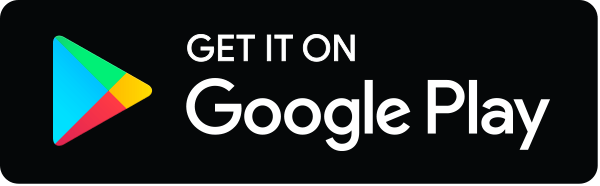