Alkaline phosphatase
Carbonic anhydrase
Superoxide dismutase
Superoxide dismutase
Energy balance
Energy in the body is obtained by breaking down the larger molecules of digested food or alternatively using stored carbohydrate, fat or protein from body reserves. The degradation of these larger molecules is referred to as catabolism, and it is accompanied by the release of energy as the chemical bonds are broken. The energy released is either used to perform work or appears as heat. Approximately 60% of the energy released during catabolism appears as heat; only 40% produces useful work. The work done may be external work, such as that performed by skeletal muscles. Alternatively, internal work may be done, either mechanically, as in cardiac contraction, or biochemically, in the synthesis of larger molecules and high-energy compounds such as adenosine triphosphate (ATP). Cellular processes, such as the active transport of substances across membranes and mucosa, also use energy internally.
The energy balance in the body can be summarised as:
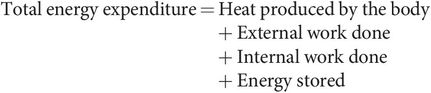
Basal metabolic rate (BMR)
The energy requirements of an individual are met by internal metabolism and reflected by total heat production (thermogenesis). The metabolic rate is the energy used by an individual per unit time, a measurement of power (kcal h−1 or watts).
BMR is dependent on various factors, which include:
Age (increased in childhood and declines with age)
Sex (testosterone causes increase)
Height, body weight and body surface area (core body temperature more effectively maintained in obesity, therefore lower BMR)
Pregnancy, menstruation and lactation (all cause increase in BMR)
Body temperature or environmental temperature (increase of 1 °C causes 10% increase in BMR)
Muscular activity
Emotional state (anxiety and pain increase BMR, depression lowers it)
Circulating levels of hormones, e.g. thyroxine and adrenaline
Recent ingestion of food (protein-rich meal raises BMR more than lipid-rich content)
Conscious level
Presence of sepsis or other disease (uncontrolled diabetes can increase BMR)
Malnutrition (causes decrease in BMR)
The basal metabolic rate (BMR) is the total energy expended over 24 hours, by a subject under standardised conditions at mental and physical rest, in a comfortable environmental temperature and fasted for 12 hours. For an average young adult the BMR is approximately 2000 kcal 24 h−1 (96 watts).
Measurement of BMR
Under the specified steady-state conditions BMR can be measured directly, using a whole-body calorimeter. The subject is placed in a calorimeter chamber, and the heat produced causes a temperature rise in a steady flow of water through the calorimeter.
The BMR can be estimated indirectly by measuring the oxygen consumption of a subject at rest. The oxygen consumption per hour is multiplied by 4.8 kcal of heat produced per litre of oxygen, to give the heat produced per hour. This is an empirical figure representative of heat production regardless of substrate used. The oxygen usage can be measured using a modified spirometer containing oxygen and a carbon dioxide absorber.
Respiratory quotient (RQ)
The respiratory quotient is a dimensionless number used in calculations of BMR. It is the ratio, at steady state, of CO2 expired to O2 consumed. The energetic equivalent of O2 is the amount of energy released from food for each mole of O2 consumed, and this varies for different substrates (Figure 21.2). The RQ of individual organs is of interest in drawing inference about the metabolic process occurring within them. For example, the RQ for the brain is regularly 0.97–0.99, indicating that its principal (but not its only) fuel is carbohydrate.
Nutrient | RQ | Calorie equivalent of oxygen (kcal L–1 O2) |
---|---|---|
Glucose | 1 | 5.01 |
Fat | 0.7 (pure fat) | 4.7 |
Protein | 0.8-0.9 | 4.6 |
Ethyl alcohol | 0.66 | 4.86 |

The volume of CO2 expired can vary with various non-metabolic states, such as hyperventilation following exercise or compensation for metabolic acidosis, which in turn will increase the RQ.
Metabolism
This term is used to describe the complex mass of biochemical reactions which break down the absorbed products of digestion to extract chemical energy, synthesise substances for structural maintenance and growth, and synthesise or detoxify waste products.
Organisation
The organisation of metabolism can be visualised as being composed of three sets of interlinked pathways. These deal with the three main types of molecule fed into the system, and are often referred to separately as carbohydrate metabolism, protein metabolism and fat metabolism. The three areas of metabolism are linked by the main energy-producing machinery, which comprises the citric acid cycle and the oxidative phosphorylation cascade (Figure 21.3).
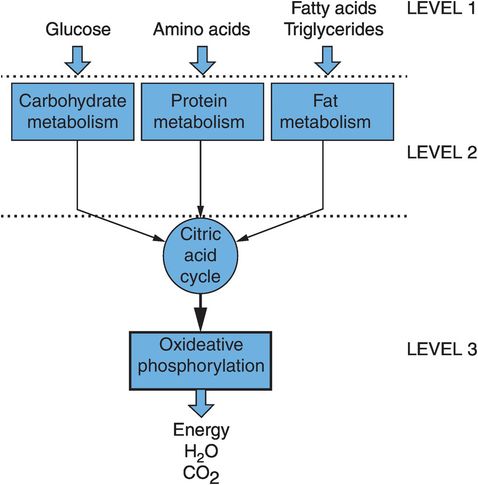
Organisation of metabolism
The extraction of energy is a primary function of metabolism and provides the driving force that determines the direction taken by the different biochemical pathways. The universal energy currency used to move and supply energy to various pathways is a high-energy compound, adenosine triphosphate (ATP). Figure 21.3 also shows how there are three levels of reactions involved in harvesting energy:
Level 1 – breakdown of carbohydrates, proteins and fats into their simple molecules of glucose and other sugars, amino acids, and fatty acids and glycerol. No energy is produced at this level.
Level 2 – degradation of these simpler molecules to a common smaller molecule, acetyl coenzyme A (acetyl-CoA). A small amount of ATP is produced at this stage.
Level 3 – passage of acetyl-CoA through the citric acid cycle, and an oxidative phosphorylation pathway, which generates > 90% of the finally harvested ATP.
In general terms, metabolism tends to proceed spontaneously in the direction of catabolism, oxidation and the production of protons. The processes and reactions reversing these trends – i.e. anabolism, reductive reactions and the maintenance of acid–base balance – require energy. Thus, the supply of chemical energy for these reactions is the key to maintaining the life process.
Chemical energy is supplied in different forms, which are outlined below.
Adenosine triphosphate (ATP)
The majority of reactions and processes requiring chemical energy to drive them use it in the form of ATP. This compound is used in processes such as the myosin–actin interaction in muscle or the active transport of substances across cell membranes, and is sometimes referred to as the common energy currency of metabolism. ATP delivers its energy when it is hydrolysed to adenosine diphosphate (ADP), since its usable energy is carried in a high-energy phosphoryl bond (Figure 21.4). The ADP is ‘recharged’ by various reactions including the citric acid cycle, oxidative phosphorylation and phosphorylation by creatine phosphate. There is a continuous turnover of ATP, and at rest an adult can use 40 kg ATP in 24 hours.
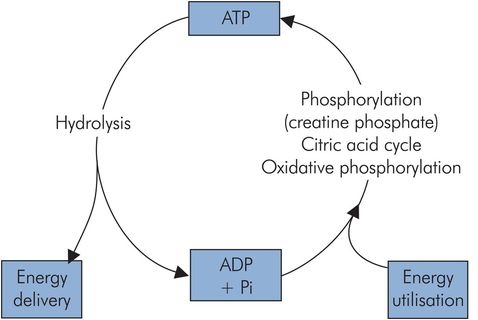
ATP cycle
Activated carriers
Although ATP has a universal role in supplying energy to drive reactions, other compounds also have the ability to drive reactions that require energy. The energy in these cases may be carried in the form of a high-potential electron or an activated group. In the case of an electron carrier, donation of an electron to one of the reagents constitutes a chemical reduction, which reverses the spontaneous tendency of the metabolic pathways towards oxidation. ATP can be regenerated with the use of a major electron carrier, nicotinamide adenine dinucleotide (NADH). Figure 21.5 shows some of the known activated carriers operating in metabolic pathways.
Carrier molecule | Group carried |
---|---|
Adenosine triphosphate (ATP) | Phosphoryl |
Nicotinamide adenine dinucleotide (NADH) | Electrons |
Nicotinamide adenine dinucleotide phosphate (NADPH) | Electrons |
Flavine adenine dinucleotide (FADH2) | Electrons |
Co-enzyme A | Acyl |
Thiamine pyrophosphate (TPP) | Aldehyde |
Creatine phosphate | Phosphoryl |
Control of metabolism
Metabolic pathways are carefully regulated, to ensure that the production of energy and intermediates meets the needs of the individual cell. The control of metabolic pathways must be flexible enough to enable adaptation to varying conditions, such as periods of starvation, exercise and stress.
The main mechanisms of metabolic control
Availability of substrate
Allosteric control of enzymes
Hormonal control
Metabolic reactions are regulated via three basic mechanisms:
The availability of substrates – Substrate levels intracellularly can be controlled by hormones that affect the transport of substrates across cell membranes. An example of this mechanism is the action of insulin in promoting glucose entry into cells.
Enzyme activity and allosteric control – An allosteric modulator binds to a regulatory site on an enzyme. This is distinct from the catalytic site. A positive allosteric modulator increases enzyme activity and stimulates the pathway, e.g. 2,3-DPG. A negative allosteric modulator inhibits the pathway (see Chapter 10, Figure 10.25).
Hormonal control – Various hormones such as the catecholamines, insulin, cortisol and thyroxine produce their physiological responses through wide-ranging changes in the metabolism of different tissues. Some examples are outlined below, while a summary of endocrine effects on metabolism is given in Chapter 22.
Additional control of metabolism is provided by maintaining separate synthetic and degradative pathways. This may be achieved by physical separation of the pathways, as in intracellular compartmentation. In such a case, for example, fatty acid breakdown (β-oxidation) occurs in mitochondria while fatty acid synthesis takes place in the cytoplasm (Figure 21.6).
Reaction site | Reaction pathway |
---|---|
Cytoplasm | Glycolysis Pyruvate oxidation Glycogenolysis Fatty acid synthesis |
Mitochondria | Citric acid cycle Oxidative phosphorylation β-oxidation |
Systemic control of metabolic responses is mainly mediated by hormones. Various hormones such as the catecholamines, insulin, cortisol and thyroxine produce their physiological responses through wide-ranging changes in the metabolism of different tissues. Examples include insulin, glucagon, adrenaline and noradrenaline (see below). A summary of endocrine effects on metabolism is given in Chapter 22.
Insulin
Insulin secretion is stimulated by glucose and amino acid uptake as well as by parasympathetic innervation. Within the liver it increases glycogen synthesis, prevents gluconeogenesis and stimulates the glycolytic production of fatty acid precursors, with resultant increase in fat storage. In the gut it increases the uptake of branched-chain amino acids, and is a stimulant to the formation of protein.
Amylin, co-secreted with insulin, may promote lactate transfer back to the liver, and support generation of fat stores.
Glucagon
Glucagon is released by the pancreas in response to hypoglycaemia. It acts on the liver to inhibit glycogen synthesis and promote gluconeogenesis and glycogen breakdown. In adipose tissue it results in the activation of lipases and fatty acid mobilisation.
Carbohydrate metabolism
Carbohydrate metabolism is mainly concerned with the generation of energy and the storage of carbohydrate as glycogen. The transportable form of carbohydrate throughout the body is the hexose sugar glucose (six-carbon, termed C6), which can be thought of as a universal fuel for all cells. The circulating levels of this are derived from:
Dietary intake of carbohydrate
Breakdown of stored carbohydrate in the form of glycogen, i.e. glycogenolysis
Synthesis from smaller precursor molecules derived from other pathways, i.e. gluconeogenesis
The energy produced by the metabolism of one mole of glucose can be measured by the number of moles of ATP produced. Under aerobic conditions there is a net gain of 38 moles ATP per mole glucose. Each ATP molecule provides the energy from one activated phosphoryl group (7.6 kcal), which gives a total yield of 288 kcal chemical energy per mole glucose. If 1 mole glucose undergoes complete combustion in a calorimeter, it liberates about 686 kcal heat. The efficiency of carbohydrate metabolism is therefore 42%.
The main pathways in carbohydrate metabolism
Glycolysis
Gluconeogenesis
Glycogenolysis and glycogenesis
Pentose phosphate pathway (PPP) or hexose monophosphate (HMP) shunt
These pathways and their relationship to each other are illustrated in Figure 21.7, and their functions are outlined below.
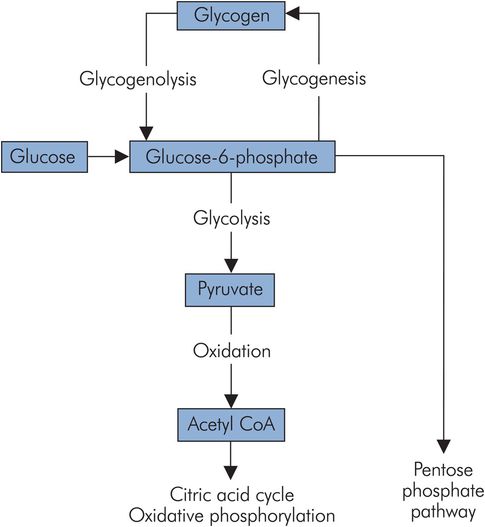
Overview of carbohydrate metabolism
Glycolysis
Glycolysis (also called the Embden–Meyerhof pathway) breaks down glucose (C6) to the triose, pyruvate (C3). Its main function is to produce pyruvate for oxidation to acetyl-CoA to feed the citric acid cycle. Glucose is activated to glucose 6-phosphate to enter the pathway. This activated form may also be utilised to generate glycogen or for conjugation, but most enters into glycolysis (Figure 21.8).
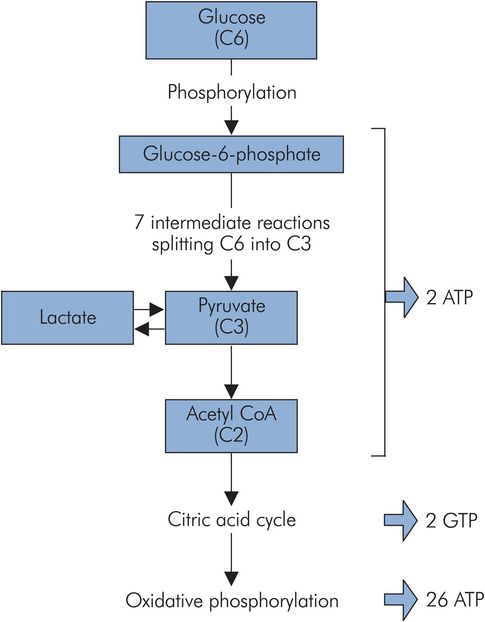
Glycolysis
This pathway uses up 2 ATP, but generates 4 ATP and 2 NADH per molecule of glucose. Thus overall there is a net gain of 2 ATP and 2 NADH. Under aerobic conditions, the 2 NADH can be oxidised through oxidative phosphorylation to generate further ATP.
Glycolysis can also proceed under anaerobic conditions, but the net energy gain is then only 2 ATP, and lactate accumulates. Anaerobic glycolysis is important to the white muscle fibres of skeletal muscle. These are capable of intense activity disproportionate to their oxygen supply, which incurs an ‘oxygen debt’ in the form of the accumulated lactate. Lactate itself can be used as a substrate by some tissues. Pyruvate is oxidised to acetyl-CoA, which enters the citric acid cycle (also known as the Krebs cycle, after the biochemist who elucidated it) or can be used in other pathways.
Rapoport–Leubering shunt
The Rapoport–Leubering shunt is active in red blood cells and produces 2,3-diphosphoglycerate (2,3-DPG) from the 1,3-DPG produced in the main glycolytic pathway. There is no net increase in production of ATP from this step, but the 2,3-DPG produced has an important allosteric effect. It fits into the space between the two β chains of deoxygenated haemoglobin and has a stabilising effect on the molecule, reducing its affinity for oxygen. This is important in enhancing the ‘offloading’ of oxygen in adaptation at altitude or during exercise, and for smokers or COPD sufferers.
Increased affinity for oxygen occurs in fetal haemoglobin, which does not have any β chains and therefore a low affinity for 2,3-DPG. Stored blood without additives (e.g. saline, adenine, glucose and mannitol) would have a low concentration of 2,3-DPG and therefore a high affinity for oxygen. Such red blood cells when transfused would have a reduced ability to offload oxygen.
Glycogenolysis
Glycogen is a branched polymer of glucose and is how carbohydrate is stored in the body. Total body reserves are about 325 g, and these are distributed between skeletal muscle and liver in the ratio of 3:1. The pathways for the breakdown of glycogen (glycogenolysis) and its synthesis (glycogenesis) are different, employing different enzymes and controls. They are illustrated in Figure 21.7. In glycogenesis glucose is activated by phosphorylation and combination with uridine triphosphate. It is then added to a pre-existing glycogen chain by glycogen synthetase. Branching of the glycogen chains requires a branching enzyme. Glycogenolysis requires a phosphorylase to activate and split off the terminal glucose unit from a glycogen chain. A debranching enzyme is also required to deal with branching points in the glycogen polymer. The enzymes in the glycogenesis and glycolytic pathways are distinct and respond individually to hormonal control. Storage of glucose as glycogen is highly efficient. The energy cost of storage and retrieval is a little over 3% of the total energy available from glucose.
Gluconeogenesis
Gluconeogenesis is the generation of glucose from substrates such as pyruvate and lactate. These in turn may be produced from amino acids by deamination, and consequently muscle mass also serves as a large potential glucose source. This occurs predominantly in the liver, and to some extent in the renal cortex. The much smaller mass of the kidney means that the overall renal contribution is small. Through gluconeogenesis plasma glucose levels can be maintained for those tissues that preferentially use glucose as an energy source. An outline of the gluconeogenesis pathway is shown in Figure 21.9. Gluconeogenesis from pyruvate is not simply a reverse of glycolysis, since the energetics so greatly favour pyruvate formation from the breakdown of glucose. Instead, pyruvate is converted to oxaloacetate at the cost of a single ATP by pyruvate carboxylase, and then phosphorylated and decarboxylated using guanosine triphosphate (GTP) as an energy source, to give phosphoenolpyruvate. The net cost of glucose synthesis from pyruvate is 6 ATP, whereas only 2 ATP are generated in glycolysis.
Pentose phosphate pathway
The pentose phosphate pathway (PPP) or hexose monophosphate (HMP) shunt is an alternative pathway for the activated form of glucose, glucose 6-phosphate. It is important in tissues that require reductive power for anabolic processes such as cell membrane repair, the synthesis of amino acids, fatty acids and steroids, and the production of nucleic acids.
The PPP is a cyclic pathway that takes in activated glucose units and produces CO2, ribose 5-phosphate and NADPH (nicotinamide adenine dinucleotide phosphate). The NADPH is important as an activated reducing agent in certain tissues such as the liver, adipose tissue, erythrocytes and the testes.
The cyclic process involves the transfer of a C2 fragment between pentoses (C5), and also provides a source of ribose 5-phosphate, an aldopentose required in the production of nucleic acids (Figure 21.10).
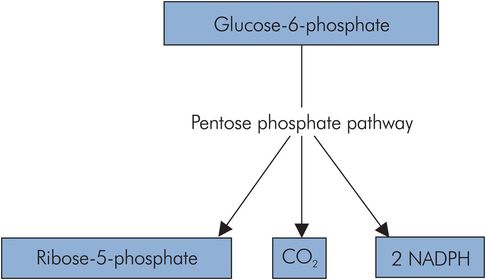
Pentose phosphate pathway
Oxidation of pyruvate to acetyl-CoA
This step in carbohydrate metabolism occurs inside the mitochondria, and it is important because it irreversibly funnels pyruvate (C3) into the citric acid cycle. The net reaction is:
It is a complex reaction requiring pyruvate dehydrogenase (an enzyme complex composed of three different types of enzyme) and several cofactors (including TPP and NAD). This key reaction is subject to feedback control, in which the presence of high levels of energy in the form of NADH, ATP and acetyl-CoA ‘switch off’ the pyruvate dehydrogenase complex. There is thus a net energy gain in this step in the form of NADH, which can be converted to ATP through oxidative phosphorylation.
Citric acid cycle
The citric acid cycle and the oxidative phosphorylation process both form the core of the energy-producing machinery in metabolism. They take place in the mitochondria and are not exclusive to carbohydrate metabolism, but form a common end pathway for the products of carbohydrate, lipid and protein metabolism. Carbohydrate metabolism and the breakdown of lipids feed acetyl-CoA into the cycle, while protein metabolism can feed into the cycle via several intermediates, including oxaloacetate (C4), α-ketoglutarate (C5) and fumarate (C4).
The main entry to the citric acid cycle is via acetyl-CoA, which is essentially an activated C2 (acetyl) group bound to a carrier (coenzyme A). This C2 fragment is loaded onto a C4 molecule (oxaloacetate) to form citrate (C6), which passes around a cycle of intermediate compounds. Two decarboxylation reactions take place to regenerate the oxaloacetate (C4).
Energy production from each citric acid cycle
Three NADH
One high-energy phosphoryl bond in GTP
One FADH2
The NADH and FADH2 are high-potential electron carriers, and enter the oxidative phosphorylation process to generate ATP (Figure 21.11).
Oxidative phosphorylation
Oxidative phosphorylation is the process of generating ATP by passing high-potential electrons carried by NADH and FADH2 down through the low-potential carriers of the respiratory chain on the inner mitochondrial membrane. High potential refers to the tendency for electrons to be transferred from these activated carriers to a cascade of lower-potential carriers (NADH-Q reductase, cytochrome reductase and cytochrome oxidase). These mitochondrial membrane carriers are basically proton pumps activated by the flow of electrons through them. They pump H+ out of the inner mitochondrion to give an H+ gradient across the inner membrane. This H+ gradient then generates ATP by driving H+ back across the inner membrane through channels of ATP synthase. The passage of H+ through these channels catalyses the synthesis of ATP (Figure 21.12).