Chapter Outline
Physical Principles of Medical Gases 10
Medical Gases 11
Medical Gas Supply and Storage 15
Medical Gas Cylinder Safety 17
Medical Gas Pipeline Network and Manifold 18
Medical Gas Delivery to the Anesthesia Machine 19
Medical Gas Removal and Waste Gas 20
Vacuum 20
Scavenging Systems 21
Conclusion 21
In the United States, the supply and sale of medical gases and medical gas delivery systems are regulated by the Food and Drug Administration (FDA). Most other industrialized nations also regulate gases used for medicinal purposes—including Canada (by Health and Welfare Canada), the United Kingdom (by the Medicines and Healthcare products Regulatory Agency), and the European Union. Requirements for the manufacturing, labeling, filling, transportation, storage, handling, and maintenance of cylinders and containers for the storage of medical gases have been published by the U.S. Department of Transportation. The Department of Labor and the Occupational Safety and Health Administration (OSHA) regulates matters affecting safety and health of employees in all industries, including employee safety when dealing with waste anesthetic gases. Other safety measures, either voluntary or regulated, are published by The National Fire Protection Association (NFPA), the Compressed Gas Association (CGA), Canadian Standards Association (CSA), and the International Standards Organization (ISO).
While regulatory measures are designed to ensure the safe and consistent manufacturing and use of medical gases, occasional accidents have been reported during their delivery. Unfortunately, these incidents have the potential to harm both patients and health care providers alike, especially anesthesiologists. Therefore proper precautions should be taken and backup systems must be put into place to minimize the impact of an adverse event. While regulatory measures play a large part in ensuring the safety supply of medical gases, perhaps even more important is the vigilant anesthesia provider, who should always be mindful of medical gases and their safe delivery.
Physical principles of medical gases must be considered by anesthesia providers as each gas has its own unique properties, which can affect storage, delivery, and use. Medical gases may be found throughout the hospital, especially in anesthetizing locations such as operating rooms. Anesthesia providers must be aware of the sources of medical grade gas to ensure an adequate supply when delivering to patients. The three most common medical grade gases (oxygen, nitrous oxide, and air) are typically supplied via a large central source. Alternatively, these and other gases may be supplied via gas cylinders, most often “E” size cylinders mounted on the anesthesia machine. A waste anesthetic gas (WAG) scavenging system and a medical suction system for surgical and anesthetic use are also provided centrally.
Physical Principles of Medical Gases
Common Gas Laws
Medical gases may be stored either as liquefied gases (oxygen, nitrous oxide, carbon dioxide) or compressed gases (oxygen, air). The state in which a gas may be stored is dependent on the physical properties, and the relationship between pressure, volume, and temperature. These relationships are described by the Common Gas Laws ( Table 2–1 ), also see chapter 27 . Although the SI unit of pressure is the pascal (see Appendix I ), anesthesiologists often measure and report pressure as kilopascals (kPa), centimeters of water (cm H 2 O), pounds per square inch (psi), or millimeters of mercury (mm Hg). 1 kPa = 7.5 mm Hg and 1 mm Hg = 1.35 cm H 2 O.
Gas Law | Formula | Relationship |
---|---|---|
Boyle’s law | P 1 V 1 = P 2 V 2 | Pressure and volume |
Charles’ law | V 1 /T 1 = V 2 /T 2 | Volume and temperature |
Gay-Lussac’s law | P 1 /T 1 = P 2 /T 2 | Temperature and pressure |
Ideal gas law | PV = nRT | Pressure, volume, and temperature |
Critical Pressure and Critical Temperature
Critical pressure and critical temperature are two important concepts that impact how medical gases are stored (also see, chapter 27 ). The critical temperature of a gas is defined as the temperature above which a particular gas is unable to be liquefied through the application of pressure. The critical pressure of a gas is defined as the pressure where a gas is able to be liquefied at the critical temperature of that particular gas.
The critical temperature of oxygen is −118° C, and, therefore, oxygen exists as a compressed gas at room temperature. The critical temperature of nitrous oxide is 36.5° C and therefore at room temperature nitrous oxide exists as a liquefied gas. To create liquid oxygen, one must first cool the oxygen below its critical temperature and then pressurize it. To maintain oxygen as a liquid, highly specialized containers, which are insulated and refrigerated, must be used.
Medical Gases
Oxygen
Medical grade oxygen is at least 99% pure. The commercial synthesis of oxygen begins with the liquefaction of compressed air. Factional distillation is then used to separate oxygen from liquid air by taking advantage of the differences in the boiling points of oxygen and nitrogen. During this process, nitrogen evaporates, first leaving liquid oxygen behind, which can then be evaporated and collected. In the medical environment, oxygen can be supplied as either a compressed gas from room temperature cylinders or as liquid oxygen from a cryogenic liquid system (CLS) container ( Figure 2–1 ). Although the systems required to supply and store liquid oxygen are more involved and expensive than regular oxygen cylinders, they often are more economical in facilities in which higher volumes of oxygen are used. This is because liquid storage is less bulky and less costly than the equivalent capacity of high pressure gaseous storage.

Liquid Oxygen Storage Systems
A typical cryogenic liquid oxygen storage system consists of one or more cryogenic storage tanks ( Figure 2–1 ), one or more vaporizers, a pressure control system, and the piping necessary to support all of the requisite fill, vaporization, and supply functions ( Figure 2–2 ). As previously mentioned, liquid oxygen must remain below its critical temperature of −118° F and pressurized to remain as a liquid. Because the temperature gradient between liquid oxygen and the surrounding environment is significant, keeping liquid oxygen well insulated from the surrounding heat is critically important. To accomplish this, cryogenic tanks are constructed in principle like a thermos bottle to shield the inner vessel from ambient heat. Vaporizers attached to the system convert liquid oxygen into a gaseous state. Downstream, a pressure control manifold then adjusts the gas pressure that is provided to the outgoing pipelines. Most cryogenic liquid systems include a backup system, which often consists of another smaller sized liquid oxygen container or a separate manifold of oxygen cylinders. In the event of an oxygen supply failure, anesthesia providers may be required to play a critical role in ensuring patient safety.

Oxygen Concentrators
Oxygen concentrators, which can generate pure oxygen from atmospheric air, are occasionally used as the primary oxygen source in some remote locations. These devices work through the adsorption of atmospheric nitrogen by a molecule sieve. To ensure adequate delivery of oxygen, the oxygen output concentration should be carefully monitored when these devices are used. Most include a pressurized reservoir, which allows a stable supply of oxygen, even when demand on the system peaks. The size of the adsorption bed is the main determinant of the maximum output of the device, and a number of different sized oxygen concentrators are commercially available. Remote military bases may use large industrial-sized units, which can supply enough oxygen for an operating room, whereas smaller portable units ( Figure 2–3 ) are often used to provide home oxygen therapy.

The Dangers of Oxygen
Although oxygen is essential for life, the anesthesia provider must be aware of potential toxicities of oxygen. Exposure to high fractional concentration of oxygen for an extended time may lead to lung injury via hyperoxia-induced necrosis and apoptosis. Oxygen is also extremely flammable and can act as an oxidizer for operating room fires. A change in oxygen concentration from 21% to 23% poses a fire threat even for substances that are not normally considered flammable, which reinforces the importance of adequate ventilation. Liquid oxygen can be splashed on items such as clothing, making them a fire hazard. Liquid oxygen may also cause freeze burns on direct contact, as it is −118° C. Nevertheless, oxygen is an essential drug used by every anesthesiologist. The physical properties of oxygen are summarized in Table 2–2 .
Oxygen | Nitrous Oxide | Carbon Dioxide | Xenon | Nitric Oxide | Carbon Monoxide | Helium | |
---|---|---|---|---|---|---|---|
Physical state in cylinder | Gas | Liquid | Liquid | Gas | Gas | Gas | Gas |
Molecular weight | 32 | 44 | 44 | 131 | 30 | 28 | 4 (He) |
Melting point (°C) | N/A | –90.81 | –56.6 | –112 | –164 | –205 | N/A |
Boiling point (°C) | –183 | –88.5 | –78.5 ∗ | –108 | –152 | –192 | –269 |
Clinical temperature (°C) | –118.4 | 36.4 | 30 | 16.6 | –93 | –140 | –268 |
Relative density, gas (air=1) | 1.04 | 1.5 | 1.52 | 4.5 | 1 | 1 | 0.14 |
Relative density, liquid (water=1) | N/A | 1.2 | 0.82 | N/A | 1.3 | N/A | N/A |
Vapor pressure at 20°C (bar) | N/A | 50.8 | 57.3 | N/A | N/A | N/A | N/A |
Solubility [in] water (mg/mL) | N/A | 2.2 | 2000 | 644 | 67 | 30 | 1.5 |
Appearance/Color | Colorless | Colorless gas | Colorless gas | Colorless gas | Colorless gas | Colorless gas | Colorless gas |
Odor | None | Sweetish | None | None | Pungent | None | None |
Other data | Gas/vapor heavier than air. May accumulate in confined spaces, particularly at or below ground level | Gas/vapor heavier than air. May accumulate in confined spaces, particularly at or below ground level | Gas/vapor heavier than air. May accumulate in confined spaces, particularly at or below ground level. Note that due to its density, Xe will flow through standard flowmeters more slowly. A conversion factor of 0.468 should be applied | Currently supplied in N 2 at less than 1000 ppm | Currently only supplied at mixtures of less than 0.3% in air and helium | Use the appropriate conversion chart when using oxygen flowmeters |
Nitrous Oxide
Joseph Priestley first described nitrous oxide preparation in 1772, and it is still prepared using the same principles. Nitrous oxide is synthesized commercially by heating ammonium nitrate and separating nitrous oxide from other compounds such as nitric oxide. Nitrous oxide exists primarily in the liquid phase at room temperature, and it is typically stored in large H-cylinders, which are cross-connected via an auto-switching manifold. Nitrous oxide storage banks typically have a smaller number of cylinders compared with oxygen supply systems because of the lower overall consumption of nitrous oxide and the higher content of liquefied gas. Nitrous oxide is often also found on the anesthesia machine in smaller E-cylinders. Because pressure within a storage cylinder remains stable around 745 psig at constant temperatures, in order to determine the amount of nitrous oxide left inside a given cylinder the cylinder must be weighed and compared against its tare weight. As liquid nitrous oxide is removed from a cylinder and approaches its critical temperature of 36.5 ° C, nitrous oxide reverts to the gaseous form. As nitrous oxide vaporizes from liquid to gas, heat is absorbed from the surrounding environment, which can lead to the formation of frost on the outside of the metallic gas cylinder.
Nitrous oxide has been used in clinical anesthesia for more than 150 years, although in the last 50 years there has been increasing concern over potential toxicity to patients, anesthesia providers, and the environment. Some choose to avoid nitrous oxide in patient care over concerns of postoperative nausea and vomiting, infection, pulmonary complications, endothelial dysfunction, and air-space expansion. Other concerns relate to the potential occupational hazards to anesthesia providers—because levels in operating rooms have occasionally been found to measure above national guidelines. Main occupational concerns include vitamin B 12 depression, genetic damage, and reproductive compromise, although there is no definitive evidence for any of these side effects among hospital workers or anesthesiologists. Finally, nitrous oxide is also known to be a potent greenhouse gas, and some have discussed the possibility of nitrous oxide contributing to global warming. The physical properties of nitrous oxide are summarized in Table 2–2 .
Medical Air
Air is a nonflammable, colorless, odorless gas that makes up the natural atmosphere of the earth. Air consists primarily of nitrogen, oxygen, and water vapor—with small amounts of carbon dioxide and other elements mixed in. Medical air may be supplied from either a central plant or a series of cylinders connected by an autoswitching manifold. Hospitals with a central plant typically have two compressors to ensure that the supply is not interrupted during service or maintenance. Having two compressors also allows the system to handle periods of peak demand. An air intake first brings air through a series of filters into a compressor. Most systems include an air cooler to cool the compressed air. From the compressor, air then passes through a one-way valve into a reservoir, where a constant pressure is maintained. Upon leaving the reservoir, another series of filters and separators removes particulate matter and impurities such as oil droplets from the pressurized air supply. A set of driers containing chemical desiccant then eliminates excess humidity from the air and a final bacterial filter removes any contaminants.
Entonox
Entonox is a 50:50 mixture of nitrous oxide and oxygen that is stored at a pressure of around 2000 psig in metallic cylinders. While used mostly for dental and obstetric analgesia, dressing changes, and transport of patients with long bone fractures, some recent studies have suggested broadening its use for procedures such as bone marrow biopsy and extracorporeal shock wave lithtripsy. Entonox is typically self-administered to patients while under medical supervision via a two-stage pressure regulator that is connected to a demand valve. Because Entonox is a mixture of gases, although the critical temperature of nitrous oxide is 36.5° C, nitrous oxide usually remains in gaseous phase. At temperatures below −5.5° C (the pseudocritical temperature), it is possible for a liquid phase to form below the gas containing 80% nitrous oxide and 20% oxygen. Because of this phenomenon, Entonox cylinders are typically stored in environments where the temperature is greater than 10° C to prevent the administration of a hypoxic mixture of gases.
Anesthesiologists should be aware of the potential for spreading infectious and/or communicable diseases via the Entonox apparatus should it be connected to the anesthesia machine. Because of the potential for cross-infection, a new Entonox apparatus must be used with each patient or a special antimicrobial filter must be placed on the tubing.
Nitric Oxide
Nitric oxide, a poisonous gas whose clinical utility has only recently been established, has found increasing use for treatment of pulmonary dysfunction in the intensive care unit and operating room and is currently being studied as a possible therapeutic agent for treatment of acute myocardial infarction.
Inhaled nitric oxide (iNO) has a profound impact on pulmonary vascular tone. Although the only current Food and Drug Administration–approved use of iNO is the treatment of hypoxic respiratory failure due to pulmonary hypertension in neonates, inhaled nitric oxide is occasionally used to treat pulmonary hypertension in adults.
Nitric oxide is stored in a nonliquefied form as a gaseous blend of nitric oxide (800 ppm) and nitrogen at a cylinder pressure of 2000 psig at 21° C. Administration occurs by injecting nitric oxide into a ventilator breathing circuit via a monitoring unit (NOx Box, Nodomo unit, iNOvent), which operates at 55 to 60 psig. The usual initial dose of nitric oxide is 5 to 20 ppm. Monitoring the levels of inspired nitric oxide, nitrogen dioxide levels, and methemoglobin levels is essential because methemoglobinemia is a well-known toxicity of iNO use. Patients should be slowly weaned off inhaled nitric oxide in decrements of 5 ppm over 6 to 8 hours and under no circumstances should nitric oxide ever be abruptly discontinued. The physical properties of nitric oxide are summarized in Table 2–2 .
Heliox
Helium-oxygen mixtures (heliox) have been used for medicinal purposes for almost 100 years. Helium is an inert gas, which also has no odor, color, or taste and does not support combustion or react with biological membranes. Helium is 86% less dense (0.179 g/L) than room air (1.293 g/L). Administration may be conducted using either an endotracheal tube or face-mask with a reservoir bag.
Treatment with heliox takes advantage of the low density of helium to improve respiratory mechanics in a number of pathological states by reducing the Reynolds number associated with flow through the airways, thereby increasing laminar flow and breathing efficiency. This technique is particularly effective in obstructive conditions. In addition to increasing laminar flow, heliox also facilitates gas diffusion, which may enhance alveolar ventilation in some circumstances. Heliox has been studied and reported by some to be effective in a variety of respiratory conditions, such as upper airway obstruction, status asthmaticus, decompression sickness, postextubation stridor, bronchiolitis, ARDS, and COPD, although more recent studies failed to show an improvement in reintubation rates when using heliox. It is important to note that heliox mixtures have the potential to decrease the work of breathing in patients with increased airway resistance, but administration does not “treat” airway resistance and is not a substitution for alleviating obstruction.
Heliox is supplied in compressed medical gas cylinders, which are typically provided in sizes E, H, and G. Helium and oxygen are usually blended to provide concentrations of 80/20, 70/30, or 60/40. Gas regulators manufactured and designed specifically for the delivery of helium must be used to deliver the gas accurately and safely. The high costs and technical challenges of delivering heliox, combined with a lack of overwhelming evidence demonstrating clinical benefit, has limited everyday use of heliox. The physical properties of heliox are summarized in Table 2–2 .
Xenon
Xenon is a noble gas, sharing nontoxic and physiologically inert properties with helium. It is found in the atmosphere in a concentration of 0.00005 ppm (50 cubic meters of air contains 4 mL of xenon). Xenon is a more potent anesthetic gas than nitrous oxide with a minimum alveolar concentration (MAC) of approximately 71%. It is poorly soluble in the blood with a blood/gas distribution coefficient of 0.115, which means it has rapid onset and offset. Administration is similar to other inhaled anesthetics, but special regulators are required to accurately determine administration values, as xenon gas is approximately five times denser than air.
Xenon is a gas still in the experimental phases for medical use. Xenon has anesthetic properties, which have been known since 1939 but were not reported until 1946 when Lawrence published a paper on xenon anesthesia in mice. Since then, multiple reports of successful use as an anesthetic have been published, and many benefits over nitrous oxide have been described such as better circulatory stability, better end-organ perfusion, and reduced neurocognitive dysfunction after general anesthesia. Environmental advantages of its use have also been documented. Unlike the fluorinated hydrocarbons in use, xenon does not deplete the ozone layer.
Xenon has advantages as a noble gas anesthetic, but still is not a major part of the anesthesiologist’s armamentarium. A major prohibitive factor is the high cost associated with a complicated production and delivery process. Current cost estimates show xenon anesthesia as costing roughly 10 times as much as conventional anesthesia. Closed circuit apparatuses with unique gas detector systems have been designed as a way to recycle gas and reduce costs and may help lead to greater use of xenon as an anesthetic in the future. The physical properties of Xenon are summarized in Table 2–2 .
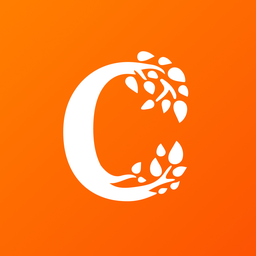
Full access? Get Clinical Tree
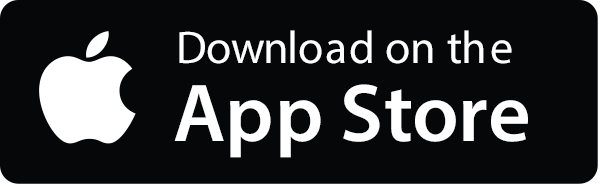
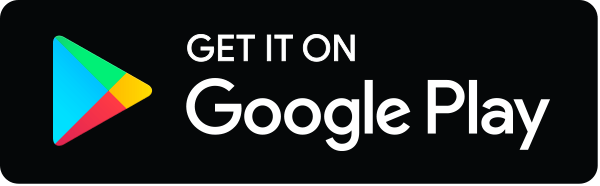