Mechanical Ventilation Part I: Invasive
Richard A. Oeckler
Rolf D. Hubmayr
Richard S. Irwin
Mechanical ventilation refers to any method of breathing in which a mechanical apparatus is used to augment or satisfy the bulk flow requirements of a patient’s breathing. Mechanical ventilation is indicated when the patient’s spontaneous ventilation is not adequate to sustain life or when it is necessary to take control of the patient’s ventilation to prevent impending collapse of other organ functions. At present, it is not known if mechanical ventilation should also be instituted to enable lung protection and prevent the potentially deleterious effects of hyperpnea in a spontaneously breathing patient with injured lungs. This chapter discusses the institution and maintenance of mechanical ventilation.
Principles of Operation
Negative-Pressure Ventilation
Until the mid-1950s, mechanical ventilators used for continuous ventilation were predominantly of the negative-pressure variety. The iron lung, or tank ventilator, was the most familiar of these. Bulk flow was mobilized into the patient’s lungs by cyclically creating a subatmospheric pressure around the chest; actually, only the patient’s head was not enclosed in the negative-pressure chamber. Subsequent ventilators applied negative external pressures to the rib cage only to induce inspiratory flow ([V with dot above]i) [1]. The original chest-enclosing ventilators of this type, called cuirass ventilators, incorporated a rigid shell that was applied to the chest. Later versions employed a much more flexible housing for the chest that was better tolerated by patients. The logistic problems encountered in providing routine nursing care for unstable patients resulted in an abandonment of negative-pressure ventilators in the acute care setting some 40 years ago. Interest in intermittent nocturnal mechanical ventilation as home therapy for chronic respiratory failure led to a minor resurgence in their use in the 1980s. However, because negative-pressure ventilators tend to be bulky, are poorly tolerated, may cause obstructive sleep apnea, and have not proved effective in the rehabilitation of patients with end-stage chronic obstructive pulmonary diseases (COPD), they have been largely replaced by positive-pressure ventilators for home use as well [2].
A recent experimental study in rabbits with injured lungs has rekindled interest in the use of negative pressure ventilators in the intensive care setting [3]. Rabbits ventilated with negative-body surface pressure had improved oxygenation and better lung recruitment than animals ventilated with equivalent amounts of positive pressure applied to the airway. The study was met with skepticism, because the results are not compatible with long established physical principles. Since the structures contained within the thorax are in essence incompressible, the findings suggest mode and instrumentation specific differences in respiratory impedance rather than inherent advantages of negative over positive pressure ventilation.
Positive-Pressure Ventilation
Positive-pressure ventilation is operative when a superatmospheric pressure is cyclically created at the upper airway. The resultant pressure gradient between the upper airway and the lungs pushes gases through the airways. In the acute care setting, positive-pressure ventilation is usually delivered through an endotracheal or tracheostomy tube. However, an increasing awareness of tube-related complications has contributed greatly to the emergence of noninvasive mechanical ventilation through a face mask, nasal mask, helmet, or mouth seal as a viable treatment option for some patients with respiratory failure (see Chapter 59 for a more complete discussion of noninvasive mechanical ventilation).
Conventional positive-pressure ventilation has come to be identified with respiratory rates up to 60 breaths per minute, even though rates above 30 breaths per minute are rarely used. Any mode of ventilation administered at higher respiratory rates is considered high-frequency positive-pressure ventilation. High-frequency oscillatory ventilation (HFOV) supports pulmonary gas exchange by entraining gas from a bias flow circuit and delivering subnormal tidal volumes (TVs) to the lungs at rates between 3 and 15 cycles per second (Hz) [4,5]. The technique was patented in the late 1950s, came to the attention of pulmonary physiologists in the 1970s, was then touted as promising treatment for babies with immature lungs, but was rejected after a large clinical trial (the HIFI study) found it to be inferior to conventional mechanical ventilation [6]. With the emergence of the “open lung concept” in the 1990s and the realization that ventilation with large TVs can injure susceptible lungs, HFOV attracted renewed interest in recent years [7,8]. In some centers, HFOV has emerged as a first-line treatment option in neonates with respiratory distress [9,10,11,12,13,14], and the MOAT trial showed a trend in favor of HFOV in adults with acute lung injury (ALI) [15]. A recent expert panel report provides detailed recommendations for HFOV [16]. Moreover, the panel identified areas for further study, such as the role of HFOV as first line treatment in adults with ALI and the choice of initial frequency settings [17]. The latter touches on unresolved issues of fundamental biologic significance: Is rate or the amplitude of lung deformation the more important risk factor for injury, and how does hypercapnia modify this risk?
Before discussing different categories and modes of positive-pressure ventilation, it is useful to review the basic mechanical determinants of patient–ventilator interactions.
Mechanical Determinants of Patient–Ventilator Interactions
Despite gross oversimplifications, linear models of the respiratory system have proved useful for the understanding of patient–ventilator interactions [18,19]. Figure 58.1 shows a simulation of volume preset (volume is the independent variable) mechanical ventilation in a linear respiratory system analogue. When ventilators are programmed to deliver a specific flow, the resulting inspiratory pressure profile contains information about the mechanical properties of the respiratory system. The pressure applied at time (t) to the tube inlet (Pi(t), near the attachment to the ventilator) is equal to the sum of two pressures, an elastic pressure (Pel(t)) and a resistive pressure (Pres(t)).

The tube outlet pressure at the junction with the balloon is equal to the pressure inside the balloon (Pel). Pres is the difference in pressure between the tube inlet and the tube outlet. Assuming linear system behavior, the inlet pressure–time profile can be computed for any piston stroke volume (Vstroke) and flow ([V with dot above]) setting, provided the resistive properties of the tube (R) and the elastic properties of the balloon (E) are known:

Elastance, E, is a measure of balloon stiffness and is equal to the ratio of Pel and Vstroke (assuming 0 volume and pressure at the beginning of balloon inflation). Therefore, Pel(t) of the first equation can be substituted with EV(t) in the second equation. Applied to the respiratory system, E reflects the elastic properties of lungs and chest wall, whereas R reflects primarily the resistive properties of endotracheal tube and airways. Because Ohm’s law states that the resistance R is equal to the ratio of pressure and flow, Pres(t) of the first equation can be substituted with the product R[V with dot above](t) in the second equation.
During inflation with constant (square wave) flow, there is an initial step change in driving pressure measured at the inlet (Pi) that equals the pressure loss across the resistive element (Pres). Thereafter, Pi increases linearly with time and volume and attains a maximal value (Ppeak) at the end of inflation. The linear rise in Pi with time (and volume) indicates that elastance of the respiratory system (Ers) is constant over the tidal breathing range and suggests that the mechanical ventilator is the only source of pressure during inflation (i.e., the respiratory muscles are relaxed).
When the airway is occluded at end inspiration, flow ([V with dot above]insp) falls to zero and the airway pressure drops from Ppeak to Pei (the end inflation/static/plateau or pause pressure). Pei represents the static elastic recoil pressure of the respiratory system at end-inflation volume (Pel). As long as Pel at end expiration is zero (absence of hyperinflation), Ers can be calculated from the ratio of Pei and tidal volume (TV).
Contrast the waveforms pertaining to volume preset mechanical ventilation in Figure 58.1 with the simulation of pressure preset (pressure is the independent variable) mechanical ventilation in Figure 58.2. When ventilators are programmed to generate a step change in pressure, the resulting inspiratory flow profile contains information about the mechanical properties of the respiratory system. Inspiratory flow rises to an early peak and then declines as the lungs fill with gas. The reason for the decline in flow with volume and time is the increase
in elastic (balloon) pressure (dashed line) (see Fig. 58.1) with volume and time. The rise in balloon pressure (surrogate for alveolar pressure, Palv) in the face of a constant Pi accounts for a progressive reduction in net driving pressure [Pi(t)– Pel(t)] during lung inflation. Because Pres varies with flow, Pres must also decline during lung inflation, reaching a minimum at end inflation. If inspiratory time is long enough to allow Pi and Palv to equilibrate (Pi = Pel), as is the case in Figure 58.2, then inspiratory flow becomes 0 and Ers may again be calculated from Pei and TV.
in elastic (balloon) pressure (dashed line) (see Fig. 58.1) with volume and time. The rise in balloon pressure (surrogate for alveolar pressure, Palv) in the face of a constant Pi accounts for a progressive reduction in net driving pressure [Pi(t)– Pel(t)] during lung inflation. Because Pres varies with flow, Pres must also decline during lung inflation, reaching a minimum at end inflation. If inspiratory time is long enough to allow Pi and Palv to equilibrate (Pi = Pel), as is the case in Figure 58.2, then inspiratory flow becomes 0 and Ers may again be calculated from Pei and TV.
The volume and flow profiles during pressure preset lung inflation are determined by the time constant of the respiratory system, which itself is a function of the respiratory system’s mechanical properties. The time constant (τ) is a feature of linear systems and defines the time it takes an elastic element to fill to approximately 63% of its capacity or conversely to passively discharge 63% of its capacity when it is exposed to a step change in pressure.

Notice from the third equation that τ is determined by the product of resistance and compliance. Because R is expressed in units of pressure × time × volume– 1 and C in units of volume × pressure– 1, their product, τ, has the units of time. In the context of pressure preset mechanical ventilation, a low value for τ predicts that airway and alveolar pressure equilibrate rapidly and that TV depends largely on respiratory compliance. Alternatively, when τ is large, TV becomes sensitive to inspiratory time and to the resistance of the intubated respiratory system (Pi > Palv at t = end inflation).
Expiratory Mechanics of the Relaxed Respiratory System
Passive expiration is driven by the elastic recoil of the respiratory system (Pel). Assuming linear pressure–volume and pressure–flow relationships, the instantaneous expiratory flow [V with dot above]exp(t) may be expressed as

Pel(t) is a function of elastance (E) (1/Compliance) and of the instantaneous volume V(t); substituting for the previous equation:

The product of R and C characterizes the time constant (τ) of single-compartment linear systems. As previously described, this represents the time at which approximately two thirds (63%) of the volume above relaxation volume (Vrel) has emptied passively.
Conventional Positive-Pressure Ventilation
Modes
The mode of mechanical ventilation refers to the characteristics of the inspiratory pressure or flow program and determines whether a patient can augment TV or rate through his or her own efforts. Descriptors of ventilation mode are conveniently separated into determinants of amplitude, rate, and relative machine breath timing.
Amplitude of Machine Output
Volume Preset Ventilation
In this mode, the machine delivers a volume set on the control panel and, within limits, delivers that volume irrespective of the pressure generated within the system (Fig. 58.2A–D). Most ventilators offer several inspiratory flow profile options that range in shape from square wave (i.e., flow remains constant throughout the inspiratory cycle) to decreasing ramp and sine wave functions. For many years, physicians have considered volume preset ventilation to be the mode of choice in the treatment of adults with acute respiratory failure because a predefined minute volume delivery is guaranteed (for exceptions, see discussion of pop-off pressures in Inflation Pressure Setting section). Yet, proponents of pressure preset modes point to several drawbacks: (a) changes in the mechanical properties of the lungs from atelectasis, edema, or bronchoconstriction may cause high inflation pressures (perhaps increasing the risk of barotrauma); and (b) changes in inspiratory effort may not result in proportional changes in ventilation. Alternatively, those who consider the avoidance of high TVs imperative for lung protection will favor volume preset over pressure preset modes [20].
Pressure Preset Ventilation
During pressure preset ventilation, the ventilator applies a predefined target pressure to the airway during inspiration (Fig. 58.2E–G). The resulting TV and inspiratory flow profile vary with the impedance of the respiratory system and the strength of the patient’s inspiratory efforts. Therefore, when either lungs or chest wall become stiff, when the airway resistance increases, or when the patient’s own inspiratory efforts decline, TV decreases. An increase in respiratory system impedance can lead to a fall in minute ventilation ([V with dot above]e), hypoxemia, and CO2 retention, but, in contrast to volume preset modes, pressure preset ventilation reduces the probability of lung injury from overdistention.
Means to Activate (Trigger) a Machine Breath
Controlled Mechanical Ventilation
Controlled mechanical ventilation is a mode during which rate, inspiratory-to-expiratory timing (I/E), and inspiratory flow (or pressure) profile are determined entirely by machine settings. Because there is never a reason to impose a rigidly set rate and breathing pattern, the term controlled mechanical ventilation usually refers to instances in which patients make no or ineffective inspiratory efforts.
Assist/Control Ventilation
The ventilator in assist/control (A/C) mode is sensitized to respond to the patient’s inspiratory effort, if present; if such efforts are absent, the machine cycles automatically and delivers a controlled breath. Therefore, a patient might conceivably assist at a rate of 12 breaths per minute although the control rate is set at 10 breaths per minute. Because volume preset mechanical ventilation had been the most widely used mode of mechanical ventilation for many years, many providers associate the A/C mode with volume preset mechanical ventilation. Nevertheless, the A/C trigger algorithm is also associated with all pressure preset modes in which pressure amplitude and timing are defined by the provider. This is the case in pressure control ventilation but not pressure support ventilation or assisted pressure release ventilation (see following discussion).
Ventilators operating in A/C mode recognize patient efforts and switch from expiration to inspiration by one of two mechanisms. During pressure triggering, phase switching occurs whenever the airway pressure falls below a predetermined level (usually 1 to 2 cm H2O below end-expiratory pressure). In this mode, a valve occludes the inspiratory port of the ventilator during expiration. An inspiratory effort against an occluded port lowers the airway opening pressure (Pao), causes the demand valve to open, and initiates a machine breath. The flow-by-trigger mode, which is available on virtually all new-generation intensive care unit ventilators, is an alternative to conventional pressure-based machine trigger algorithms [21].
During flow-by, a continuous flow of gas is presented to the patient and is vented in through the expiratory tubing unless the patient makes an inspiratory effort. This so-called base flow can be set by the operator between limits of 5 to 20 L per minute. When the patient makes an inspiratory effort(s), he or she diverts flow into the lungs, resulting in a discrepancy between base flow and the flow of gas through the expiratory circuit. The minimal difference between inspiratory and expiratory flows, which results in a machine breath, is determined by the flow sensitivity setting and can vary from 1 to 3 L per minute. Most modern ventilators combine pressure and flow-triggering algorithms so that concerns about benefits of one over the other triggering mechanism are no longer relevant.
Short-lived inspiratory efforts that occur during early expiration are often insufficient to be recognized by either pressure or flow triggering algorithms. Careful inspection of airway pressure and flow profiles, of neck and chest wall motion, or intermittent flaring of the alae nasi should alert the physician to this phenomenon, which indicates a dissociation between machine rate and the patient’s own intrinsic respiratory rate. Wasted inspiratory efforts are commonly seen in weak, sleeping, or heavily sedated patients and in patients unable to overcome intrinsic (or auto) positive end-expiratory pressure (PEEP) (see following discussion) [22].
The A/C feature has lured many physicians into the erroneous assumption that the machine backup rate setting is unimportant (see discussion on rate settings and troubleshooting in Minute Ventilation section). Although only a modest inspiratory effort is required to trigger the ventilator, many patients perform muscular work throughout the entire assisted breath in direct proportion to their ventilatory drive [23]. If the patient’s work of breathing is deemed excessive and potentially fatiguing, the physician should lower the trigger sensitivity setting, consider raising [V with dot above]i, evaluate oxygenation and alveolar ventilation, assess the adequacy of machine backup rate and PEEP settings, and address sedation and pain control.
In years past, there had been a great reluctance to use of neuromuscular blocking agents (NMB) to prevent adverse patient–ventilator interactions. However for patients with ALI or the acute respiratory distress syndrome (ARDS), who frequently
double their VT by breath stacking and are therefore at risk for ventilator associated lung injury, this reluctance may no longer be appropriate. In several randomized clinical trials, the group of Papazian has reported that patients with ALI, who were initially managed with NMB, had improved surrogate physiologic endpoints, spent fewer days requiring mechanical ventilation and were more likely to survive than those who were managed with sedatives and narcotics alone [24,25] However, the issue is far from settled, awaits independent confirmation, and importantly, the data do not apply to patient populations without acute lung injury, whose risk for ventilator induced injury is much lower.
double their VT by breath stacking and are therefore at risk for ventilator associated lung injury, this reluctance may no longer be appropriate. In several randomized clinical trials, the group of Papazian has reported that patients with ALI, who were initially managed with NMB, had improved surrogate physiologic endpoints, spent fewer days requiring mechanical ventilation and were more likely to survive than those who were managed with sedatives and narcotics alone [24,25] However, the issue is far from settled, awaits independent confirmation, and importantly, the data do not apply to patient populations without acute lung injury, whose risk for ventilator induced injury is much lower.
Intermittent Mandatory Ventilation
Early versions of the intermittent mandatory ventilation (IMV) mode combined spontaneous breathing and volume preset-assisted ventilation [26]. For example, at an IMV rate of 6 breaths per minute, the ventilator would deliver a volume preset breath every 10 seconds. Between these mechanically controlled breaths, the patient would breathe spontaneously and the ventilator would serve as a source of warmed, humidified, potentially oxygen-enriched gas. During the years, IMV has become more complex. In modern ventilators, mandatory breaths may be volume or pressure preset and it has become commonplace to augment spontaneous breaths with positive airway pressure as well (e.g., by using the pressure support mode).
Virtually all modern ventilators use synchronized IMV algorithms that prevent the patient from getting a double breath with IMV (i.e., a machine breath is delivered at the end of a spontaneous inspiratory effort). At intervals determined by the IMV frequency setting, the machine becomes sensitized to the patient’s inspiratory effort and responds by delivering a pressure or volume preset breath. Between these preset cycles, the patient breathes spontaneously (with or without pressure support) at a rate and depth of his or her own choosing. For example, at an IMV rate of 6 breaths per minute, the ventilator allows the patient to breathe spontaneously while the delivery of preset breaths is initially refractory to the patient’s efforts. After 10 seconds elapse, the machine is rendered sensitive. When an effort occurs, the ventilator delivers a preset breath and the patient breathes spontaneously until 10 seconds after the end of the previous refractory period. If the patient does not make an inspiratory effort during the sensitive period, the ventilator delivers a controlled breath after sufficient time elapses. This time varies inversely with the IMV backup rate; it is equal to 60 seconds divided by the IMV rate. In the example given here, the period would be 10 seconds (60 divided by 6).
IMV is a very complex mode with numerous degrees of freedom. It was originally introduced as a weaning modality. However, in controlled clinical trials this mode has performed inferior to other weaning techniques (see Chapter 60) [27,28,29]. Nevertheless, in many institutions IMV remains the default mode for patients who are relatively easy to ventilate. Familiarity with this mode and the high incidence of ventilator-induced apneas in sleeping or comatose patients, who are supported in modes without mandatory backup rates, are likely reasons for the persistent popularity of IMVs [30,31,32].
Pressure Support Ventilation
Pressure support ventilation (PSV) is a form of pressure preset ventilation. It is intermittent positive-pressure breathing with a sensing device that delivers the breath at the time the patient makes an inspiratory effort. As the lungs inflate, [V with dot above]i begins to decline because airway pressure and the pressure generated by inspiratory muscles are opposed by rising elastic recoil forces. When [V with dot above]i reaches a threshold value (which differs among vendors), the machine switches to expiration. Inspiratory off-switch failure, that is, application of inspiratory pressure after cessation of inspiratory muscle activity, is common during PSV [31,33]. High inspiratory pressure settings, a low respiratory drive, airflow obstruction with dynamic hyperinflation, and air leaks predispose patients to this form of patient–ventilator asynchrony [31,34]. Asynchrony, in turn, is an underappreciated cause of sleep disruption [22,35].
PSV is a popular weaning mode for adults. A review of the weaning literature (see Chapter 60) suggests that this mode is as effective as intermittent T-piece trials of spontaneous breathing in liberating patients from mechanical ventilation [36,37,38]. It should also be noted that PSV is a useful alternative to volume preset mechanical ventilation, particularly in patients with increased rate demands and respiratory drive [39]. However, the risk of lung injury from sustained increases in TV probably applies to the PSV mode as well, because airway pressure despite being low does not inform about lung stress.
Pressure Control Ventilation
Pressure control ventilation (PCV) is a form of pressure preset ventilation. It differs from PSV in two important respects: The operator sets a machine backup rate and determines inspiratory time (Ti). The A/C feature assures ventilation of the lungs in patients who are prone to apneas. Cessation of inspiratory effort can be a problem in sleeping adults who are ventilated in the pressure support mode [30,40]. On the other hand, PCV does not offer the patient the same control over TV and breathing patterns as PSV. For this reason, PCV with long Ti, is usually reserved for hypoxic heavily sedated or paralyzed patients in whom the need to match ventilator rate and timing with intrinsic respiratory rhythms is not an issue.
Assisted Pressure Release Ventilation and Bilevel Support Modes
Although bilevel positive airway pressure ventilation (BiPAP) technically describes any mode in which the pressure applied to the airway cycles between two provider set levels, in practice most associate BiPAP with a PSV like mode that is often used in noninvasively mechanically ventilated patients. It is a pressure/time cycle mode which allows the patient’s own breathing to supplement ventilator output. There are subtle differences in the cycling algorithms among devices of different vendors, somewhat clouding the literature on the topic. When bilevel pressure ventilation is delivered with an inverse inspiratory to expiratory time ratio, the mode becomes indistinguishable from assisted pressure release ventilation (APRV). Arguments in favor of bilevel pressure ventilation modes including APRV in patients with injured lungs center on improved gas exchange and maintenance of dependent lung aeration attributable to preserved diaphragm activity [41]. However, superiority of bilevel modes relative to volume preset modes has not been established. Detractors point out that it is more difficult to assure delivery of lung protective tidal volumes in pressure preset modes.
Noninvasive Mechanical Ventilation
Noninvasive mechanical ventilation (NMV) (see Chapter 59) encompasses all modes of ventilatory assistance that can be applied without an endotracheal tube. The realization that certain patients benefit from intermittent positive pressure breathing through a mask has fundamentally changed the initial management of many respiratory failure syndromes. The literature on NMV has grown exponentially, and the following comments focus on the use of NMV in the acute care setting.
Several randomized prospective clinical trials have shown NMV to be an effective initial therapy for patients with
impending or overt respiratory failure [42,43,44,45,46,47]. The early application of NMV in the emergency department is particularly important in patients with exacerbation of airways obstruction as it may spare them the risks and discomfort associated with intubation and conventional mechanical ventilation. Other conditions in which NMV appears to be an effective initial rescue treatment include ventilatory insufficiency from chest wall disease, neuromuscular weakness, and sleep-related breathing disorders. The use of NMV in hypoxic forms of respiratory failure is increasing, but in comparison to COPD its efficacy is less well established [46,48,49,50,51,52,53], NMV is relatively contraindicated in patients who cannot protect their airway or who cannot clear their secretions, and in our experience NMV invariably fails in patients with shock or metabolic acidosis [54].
impending or overt respiratory failure [42,43,44,45,46,47]. The early application of NMV in the emergency department is particularly important in patients with exacerbation of airways obstruction as it may spare them the risks and discomfort associated with intubation and conventional mechanical ventilation. Other conditions in which NMV appears to be an effective initial rescue treatment include ventilatory insufficiency from chest wall disease, neuromuscular weakness, and sleep-related breathing disorders. The use of NMV in hypoxic forms of respiratory failure is increasing, but in comparison to COPD its efficacy is less well established [46,48,49,50,51,52,53], NMV is relatively contraindicated in patients who cannot protect their airway or who cannot clear their secretions, and in our experience NMV invariably fails in patients with shock or metabolic acidosis [54].
Less Commonly Used Modes of Mechanical Ventilation
Some new-generation mechanical ventilators feature modes with closed-loop feedback control of both pressure and volume [55,56]. Dual-control modes seek to provide a target ventilation while maintaining low inflation pressures. To this end, ventilator output is adjusted based on volume, flow, and pressure feedback within each machine cycle or gradually from one cycle to the next. Modes that adjust output within each cycle execute a predetermined pressure–time program as long as the desired TV is reached. When the TV target is not reached, inspiration continues at a preselected inspiratory flow rate (volume-limited) until the target volume is attained. Volume-assured pressure support and pressure augmentation are examples of such modes. Breath-to-breath dual control modes are pressure-limited and time or flow cycled. Ventilator output is derived from the pressure–volume relationship of the preceding breath and adjusted within predefined pressure limits to maintain the target TV. Pressure-regulated volume control, volume control plus, auto-flow, adaptive pressure ventilation, volume support, and variable pressure support are examples of breath-to-breath control modes.
Neurally adjusted ventilatory assistance (NAVA) and proportional assist ventilation (PAV) are the most complex and arguably the most promising closed-loop ventilation modes [57,58]. At the time of this writing, only PAV is commercially available in the United States. During PAV, the relaxation characteristics of the respiratory system are assessed on a breath-by-breath basis so the ventilator may provide a set fraction of the inspiratory elastic and flow resistive work [57,58,59]. Its applications in NMV will be discussed in Chapter 60. During NAVA, the diaphragm’s electrical activity is recorded with an esophageal probe and the signal is conditioned and transposed into a positive airway pressure output. Preliminary observations on patients suggest that NAVA results in greater patient–ventilator synchrony than conventional modes [60]. Moreover, there is some evidence from animal models that NAVA affords greater lung protection from ventilator associated injury [61] by virtue of preserved coupling between respiratory control and motor output. At the time of this writing however, there is no evidence that either dual- or closed-loop modes are safer or more effective than conventional approaches.
Choice of Ventilation Mode
The therapeutic end points of mechanical ventilation vary considerably among different respiratory failure syndromes. For example, the ventilatory management of patients with ALI has little in common with that of patients suffering from exacerbation of COPD. However, the need for pathophysiology-based treatment objectives should not be confused with a need to find an optimal ventilation mode for each class of respiratory disorders. In general, the therapeutic goals of mechanical ventilation can be achieved with more than one mode [62].
Ventilator Settings
Fraction of Inspired Oxygen
The hazards of indiscriminate administration of oxygen to patients with CO2 retention and the topic of pulmonary oxygen toxicity are discussed in Chapters 49 and 62. Notwithstanding these very real concerns, oxygen must never be withheld from a mechanically ventilated patient. If there is any suspicion that the patient may require oxygen, it should be given. Certain drugs, such as bleomycin, may sensitize the lungs to reactive oxygen species-mediated injury and it is advisable to minimize the fraction of inspired oxygen (FIO2) in patients receiving them [63]. Adjustments in FIO2 are usually guided by pulse oximetry and/or arterial blood gas analyses. Most care-givers dose FIO2 to an arterial oxygen tension (PaO2) more than 60 mm Hg and/or an oxygen saturation more than 90%. Although these targets are based on reasonable physiologic assumptions, they are nevertheless empiric. Some accept lower O2 saturations in young patients with adequate end organ perfusion, when the treatment of hypoxemia seems risky. Ultimately, the risk associated with hypoxemia must be balanced against the risk of oxygen toxicity and the risks associated with raising PEEP and manipulating hemoglobin and cardiac output. It is currently believed that an FIO2 below 0.6 is not injurious to the lungs even when used for days or weeks. Because the contribution of oxygen to lung injury cannot be separated from that of other insults (e.g., sepsis-related inflammatory mediator release, gastric acid, infectious agents, lung parenchymal stress), oxygen dosing recommendations remain open to debate.
Tidal Volume
When using a volume preset mode, TV is either set directly or follows from the minute volume and rate setting. When a pressure preset mode is used, TV is the consequence of the patient’s respiratory effort, the mechanical properties of the respiratory system, the pressure amplitude setting, and the duration over which the inflation pressure is applied. TV is arguably the most important ventilator setting. Historically it had been common practice to scale TV to actual body weight. This practice is no longer acceptable because the high prevalence of obesity biases TV settings toward injurious levels, and because height and gender are much more powerful predictors of lung size than is body weight [64]. Height and gender are also used to estimate ideal or predicted body weight that by virtue of its use in the acute respiratory distress syndrome network (ARDS Net) trials has become the preferred TV scaling factor [65].
Predicted Body Weight (in kg):

Most experts suggest to target TV in patients with injured lungs between 6 ± 2 mL per kg predicted body weight. Although the evidence in support of lung protective TV settings in other patient population is less compelling, there is no reason to suspect that TV settings in excess of 8 ml/kg are of benefit. There is overwhelming evidence that inflating the lungs above total lung capacity (TLC) can damage normal lung units, particularly when this occurs in conjunction with large tidal excursions [66,67,68]. In patients with a normal body habitus (i.e., normal chest wall recoil and compliance), TLC corresponds to a plateau or end-inflation hold pressure between 30 and 35 cm H2O [69]. For this reason most experts limit respiratory system inflation pressures to 30 cm H2O or less, However, in light of recent data this guideline may have to be reevaluated (see discussion about inflation pressure setting later). Unless lung function is severely impaired, even large TVs are unlikely
to distend the lungs beyond their structural limit (i.e., TLC). This has caused some experts to reason that reducing TV to values less than 8 mL per kg ideal body weight (as is custom in ARDS Net trials) is neither required nor beneficial in patients with plateau pressures less than 30 cm H2O. We address this controversy in greater detail in the context of ventilator management of ARDS.
to distend the lungs beyond their structural limit (i.e., TLC). This has caused some experts to reason that reducing TV to values less than 8 mL per kg ideal body weight (as is custom in ARDS Net trials) is neither required nor beneficial in patients with plateau pressures less than 30 cm H2O. We address this controversy in greater detail in the context of ventilator management of ARDS.
Sighing and Recruitment Maneuvers
Periodic hyperinflation (the “sigh” or “yawn” maneuver) is a spontaneous reflex in conscious humans. Periodic stretching of the lung stimulates surfactant production and release and therefore prevents atelectasis [70,71]. However, the effects of sighing on mechanics and gas exchange tend to be short lived and vary with disease state, posture, and ventilator mode and setting [72,73]. Some experts recommend that the lungs of patients with ARDS should be intermittently held at high volumes and pressures (e.g., 30 to 50 cm H2O for 20 to 40 seconds) to recruit collapsed and/or flooded units [74,75,76]. The use of recruitment maneuvers has been associated with improved gas exchange, altered lung mechanics and less inflammation in experimental lung injury models [77,78,79,80,81]. By virtue of volume and time history, such maneuvers tend to potentiate the effects of PEEP on functional residual capacity [82]. Incorporating sighs into a lung-protective mechanical ventilation strategy in patients with early ALI/ARDS improved oxygenation and static compliance, but had no effect on survival [83]. This confirms that periodic lung inflation and recruitment maneuvers exert demonstrable effects on lung function, but are not appropriate surrogate markers of clinical efficacy. In fact, a post hoc analysis of the ARDS-Network low tidal volume trial revealed that patients, who had been randomized to the injurious high tidal volume arm had better oxygenation during the first 24 hours than those, who in hindsight, had received lung protection [65].
“Biologically variable mechanical ventilation” is an experimental mode of mechanical ventilation that seeks to maximize lung recruitment by preserving the normal breath-to-breath variability in TV and rate [84]. Biologically variable mechanical ventilation is superior to evenly timed sighs in improving gas exchange and lung function [85,86]. Moreover, biologically variable mechanical ventilation finds a strong mechanistic underpinning in the principle of stochastic resonance [87]. Stochastic resonance is a feature of nonlinear systems that explains why seemingly minor variability in input (e.g., TV) has major effects on output (e.g., number of recruited alveoli) [88]. At the time of this writing, this mode is not available for commercial use in North America.
Inflation Pressure Setting
Volume Preset Mode
Although pressure is a dependent variable during volume preset ventilation, generally the cycling pressure should not be allowed to increase without limit. Rather, a pressure limit or pop-off pressure should be imposed to guard against inadvertent overinflation and possible lung rupture [89]. This is set directly on the ventilator’s control panel, and when and if it is reached, a visual and possibly audible alarm alerts the attendant to the fact that the machine has popped off. That particular cycled breath will have been partially aborted and the patient will have received only part of the volume set on the control panel. A random, infrequent pop-off cycle is most often caused by the patient’s coughing or splinting, and need not be cause for concern. However, repeated popping off may be an indication that the patient is in acute respiratory distress and should prompt those in attendance to disconnect the patient from the ventilator to determine the cause of the problem. Although the patient is manually ventilated, a suction catheter should be passed through the endotracheal tube to determine whether it is patent, and the ventilator should be checked to ensure it is functioning properly. Other factors to consider are whether the patient is undersedated or anxious and in pain, whether the patient’s airway resistance has increased (e.g., bronchospasm, excessive secretions, mucus plugging), whether the endotracheal tube has migrated beyond the carina, or whether a pneumothorax has developed.
Pop-off pressures should usually be set at a level slightly above Ppeak observed during normal cycling and should not be higher than 40 cm H2O, whereas PEEP and TV should generally be set to maintain plateau pressures 30 cm H2O or less. Although no specific airway pressure is guaranteed to exclude the risk of barotrauma, higher airway pressures appear to impose an increased risk of alveolar overdistention that can lead to permeability pulmonary edema, alveolar hemorrhage, subcutaneous emphysema, pneumomediastinum, and pneumothorax. There is general agreement that the main determinant of alveolar overdistention is the end-inspiratory lung volume [67]. On the basis of this reasoning, Dreyfuss et al. [90] and Dreyfuss and Saumon [91] have coined the term volutrauma distinct from barotrauma. The term barotrauma refers to injury manifest as extra-alveolar air, whereas volutrauma denotes injury manifest as altered lung barrier function. Regardless, one should appreciate that lung stress (transpulmonary pressure) and lung volume cannot be uncoupled and that neither is routinely measured at the bedside. TV and plateau pressure, the variables that are being measured, inform only indirectly about lung volume and lung stress, and the provider must integrate them with estimates of chest wall compliance (or, more specifically, chest wall recoil) [92]. For these reasons, we believe that plateau pressure limits of 30 cm H2O should be ignored in patients with obesity, ascites, or abdominal distention [93].
There is evidence that esophageal manometry guided PEEP management is associated with improved lung function compared to a conventional ARDS-Network based approach [94]. In a small clinical trial survival trends favored esophageal manometry guided PEEP management, even though a substantial number of patients were ventilated to plateau pressures in excess of 30 cm H2O [95]. A subsequent report suggested that the majority of ARDS patients have substantially increased end-expiratory chest wall recoil pressures and that the corresponding implications for PEEP management may not be evident from airway pressure recordings alone [96]. The issue is far from settled, because of concerns for measurement bias in esophageal pressure derived estimates of transpulmonary pressure [97]. Nevertheless, a rigorous adherence to an absolute plateau pressure safety limit of 30 cm H2O in patients with ALI seems no longer advisable. There is a healthy debate about the appropriate balance between maximizing lung recruitment (through the application of PEEP) and minimizing end-inspiratory parenchymal stress (by avoiding high inflation pressures). In following this debate, we conclude that there is neither a single safe inflation pressure nor safe tidal volume threshold. Rather both surrogates of injurious stress and strain are invariably intertwined. For example, inflating the lungs to near maximal capacity during HFOV seems quite safe provided tidal volumes are kept relatively low. Alternatively, a TV which would likely be injurious during HFOV is well tolerated provided lung inflation pressure is kept relatively low.
Because of the increasing risk of barotrauma with rising airway pressures, it is important to determine not only why peak airway pressures are increasing but also to try to reduce them. For instance, if agitation is responsible, the patient should be sedated and, as addressed earlier, at times even paralyzed [24,25]. Although lower [V with dot above]i rates might help achieve the goal of decreasing peak airway pressure, it is not clear that this prevents susceptible lung units from overdistention injury. Reductions in flow without concomitant reductions in TV may simply reduce the resistive pressure that is dissipated across the endotracheal tube without lowering peak transpulmonary pressure or lung stress.
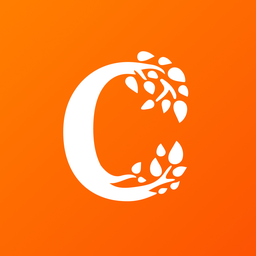
Full access? Get Clinical Tree
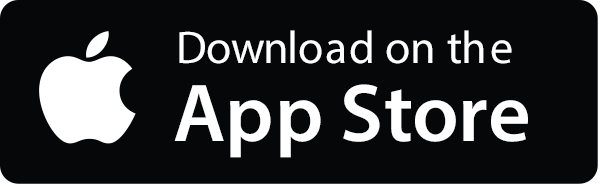
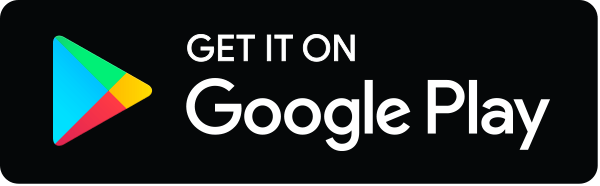
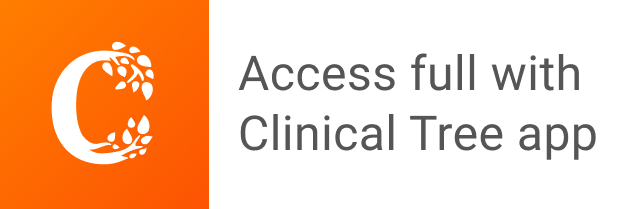