Marcelo Gama de Abreu, Jakob Wittenstein One-lung ventilation (OLV) for thoracic surgery still poses a challenge for the anesthesiologist. The presence of atelectasis not only increases the risk of intraoperative hypoxemia but also favors the inhomogeneity of ventilation during OLV, which in turn can increase the forces applied to the lung parenchyma. Those forces increase the likelihood of ventilator-induced lung injury (VILI) affecting also previously noninjured lungs. In this chapter, we will review the mechanisms of VILI, and explore the basic concepts of so-called protective ventilation during OLV for thoracic surgery, with emphasis on the maintenance of adequate gas exchange while avoiding injury that might increase the risk of postoperative pulmonary complications. one-lung ventilation; thoracic surgery; thoracic anesthesia; postoperative pulmonary complications. One-lung ventilation (OLV) was first described in 1931, and the first pneumonectomy for cancer was performed in 1933. Although technology has evolved since then, OLV still poses a challenge for the anesthesiologist. For several decades, the impairment of gas exchange during OLV represented the focus of the attention. Accordingly, intraoperative mechanical ventilation settings were aimed at avoiding or reversing hypoxemia, as well as hypercapnia, without much concern about the potentially harmful effects of the mechanical stress on the lung parenchyma. Those settings included tidal volume (VT) of 10–12 mL/kg of the actual body weight (for a single lung), and zero positive end-expiratory pressure (PEEP). Since then, however, the concept of ventilator-induced lung injury (VILI) emerged and became the cornerstone of mechanical ventilation. The so-called “lung-protective ventilation” strategy, which is based on the use of low VT with low distending pressures, gained much attention when a multicenter randomized trial showed that VT of 6 mL/kg of the predicted body weight (pbw) led to lower mortality in patients with the acute respiratory distress syndrome (ARDS) when compared with VT of 12 mL/kg pbw.1 It is worth noting that the lung-protective strategy was associated with lower oxygenation than the nonprotective strategy in that study. Furthermore, a meta-analysis showed that use of low VT is associated with favorable outcomes also in patients without injured lungs.2 The use of protective ventilation strategies is not limited to the intensive care unit setting and became widespread also among anesthesiologists to be applied in the operating room. A recent compilation of recommendations for intraoperative mechanical ventilation emphasized the role of low VT and distending pressures, as well as of adequate PEEP levels, during general anesthesia, as a means to reduce the risk of postoperative pulmonary complications (PPCs).3 However, those recommendations did not address OLV because protective ventilation during OLV has not been investigated yet with adequately powered trials.4,5 Clearly, it must be taken into account that mediastinal displacement, surgical manipulation and chest immobilization, external pressure on the dependent lung,6 and atelectasis formation increased during thoracic surgery as compared with the other types of surgeries. To date there is no convincing evidence that the data derived from patients with ARDS in the ICU can be translated to the thoracic surgical patients.7 In this chapter, we will review the mechanisms of VILI, and explore the basic concepts of protective mechanical ventilation during OLV for thoracic surgery, with emphasis on the maintenance of adequate gas exchange, while avoiding injury that might increase the risk of PPCs. During mechanical ventilation, atelectatic and overdistended lung regions may coexist, increasing vulnerability of lungs to the potentially detrimental effects of mechanical ventilation.8,9 Accordingly, the regional stress of the lungs is not well reproduced by gross measurements of lung mechanics.10,11 Different mechanisms have been proposed for VILI, most of which are interrelated (Fig. 20.1). Increased airway pressure (barotrauma) or the application of relatively high VT (volutrauma) may generate excessively high stretch (strain) and transpulmonary pressures (stress), exceeding the elastic properties of the lung parenchyma above its resting volume and causing direct physical damage of the alveolar-capillary barrier and the cells attached.12,13 Also, tidal close-and-reopening of lung units, so-called atelectrauma,14 can occur in regions close to atelectasis. Importantly, barotrauma, volutrauma, and atelectrauma can have effects on both epithelial and endothelial cells,15,16 and cause fragmentation of the fragile extracellular matrix.17,18 The mechanical damage of the extracellular matrix favors the formation of interstitial edema and activation of metalloproteinases, may not necessarily cause inflammation, however, these fragments of the extracellular matrix have the potential to activate inflammatory mediators.19,20 Furthermore, the damage of the extracellular matrix induced by mechanical ventilation might be exacerbated by fluid load,21 which is not uncommon to be underestimated during general anesthesia. In cells, especially those from the alveolar epithelium, mechanical stress can be transducted into chemical signals through different pathways, which elicit proinflammatory and profibrotic responses. This process is known as “mechanotransduction“.22 Mechanotransduction can lead to cell apoptosis, and release of homing molecules that promote homing and remote activation of neutrophils and macrophages in lungs and peripheral organs.23 These effects are termed “biotrauma“.24 In the alveolar-epithelial barrier, mechanical stress can disrupt endothelial cells and lead to capillary stress failure.25,26 Also in the presence of structurally intact cells, disruption of adherence junctions (“tight junction”) can occur.27 Together, those mechanisms increase the epithelial permeability of inflammatory factors and the formation of edema. On the alveolar side of the barrier, the permeability of the alveolar epithelium can also increase with mechanical stress.28 Those phenomena are more likely during overstretching, but have been described also during atelectrauma.29,30 Besides the increased permeability during ventilation with high VT, there is also a risk that excessive stretch impairs the Na, K-adenosine triphosphatase activity,31 further contributing to edema formation. Ventilation with low VT reduces this risk of excessive stretch, but on the other hand, it favors hypoxia, and may impair fluid clearance.32 Independently of the mechanism, alveolar edema interferes with surfactant function.33 In addition to those phenomena, translocation of pathogens and proinflammatory mediators is more likely to occur when the alveolar-capillary barrier loses integrity, which may result in damage of distal organs.34 During OLV, presence of atelectasis favors the inhomogeneity of ventilation, which in turn can increase the forces applied to the lung parenchyma.9 Those forces increase the likelihood that one or more of the mechanisms mentioned earlier takes place, and VILI can result even in previously noninjured lungs. Mechanical ventilation settings may affect the risk of VILI and subsequent development of PPCs, which increase morbidity and result in a longer duration of in-hospital stay and even increased risk of death.35–37 Therefore protective mechanical ventilation settings should be known in detail by anesthesiologists performing OLV (Table 20.1). Table 20.1 – Volume-controlled ventilation preferred to keep tidal volume constant independently of surgical manipulation; can result in higher peak pressures – Pressure-controlled ventilation preferred to keep peak pressure within safety margins, and during bronchoscopy; can result in derecruitment – Consider 6–8 mL/kg pbw when severe hypercapnia is present and in patients with COPD – Obstructive lung diseases: lower respiratory rates preferred – Consider PEEP titration according to compliance of the respiratory system or driving pressure if hypoxemia occurs – Be aware of auto-PEEP, and its potential consequences – Consider values ≤ 25 cm H2O – consider I:E 1:2 to 1:3 in case of auto-PEEP – During OLV, titration to SpO2 ≥ 92%, as individually appropriate – Potentially useful for reversal of intraoperative hypoxemia, especially if followed by titration of PEEP – Stepwise increase of VT or PEEP during tidal ventilation preferred over manual bag squeezing COPD, Chronic obstructive pulmonary disease; OLV, one-lung ventilation; SpO2, peripheral oxygen saturation; TLV, two-lung ventilation. As shown in Fig. 20.2, there are at least two possible mechanical ventilation strategies to reduce the risk of VILI, namely, the so-called open-lung approach and the lung rest strategy (permissive atelectasis). The open-lung approach is based on alveolar recruitment maneuvers (RM) (open the lungs), high levels of PEEP (keep the lungs open),38 and the use of low VT. The lung rest strategy is based on the concept that atelectatic-collapsed lung regions, when not subjected to repetitive opening and closing, are protected from inflammation,39 and aims at a minimal PEEP level to assure adequate gas exchange (oxygen saturation ≥ 88%–90%), low VTand low respiratory rate.40 In a porcine model of experimental pneumonia, both exogenous surfactant administration and ventilation according to the open-lung approach attenuated bacterial growth and systemic translocation by minimizing alveolar collapse and atelectasis formation.41 In a similar model of experimental pneumonia in mechanically ventilated pigs, bacterial translocation was lowest with individually tailored PEEP levels, whereas low and high PEEP promoted bacterial translocation.42 In isolated nonperfused mouse lungs, both an open-lung approach (VT of 6 mL/kg, RM and PEEP of 14–16 cmH2O) as well as a lung rest strategy (VT of 6 mL/kg, PEEP of 8–10 cmH2O, no RM) were associated with reduced pulmonary inflammatory response and improved respiratory mechanics compared with injurious mechanical ventilation (VT of 20 mL/kg, PEEP of 0 cmH2O).43 Interestingly, the lung rest strategy was associated with less apoptosis but more ultrastructural cell damage, most likely because of increased activation of mitogen-activated protein kinase pathways as compared with the open-lung strategy.43 In healthy mice, mechanical ventilation with a VT of 8 mL/kg and PEEP of 4 cmH2O induced a reversible increase in plasma and lung tissue cytokines, as well as increased leukocyte infiltration, but the integrity of the lung tissue was preserved.44 In another investigation, even least-injurious ventilator settings were able to induce VILI in the absence of a previous pulmonary insult in mice.45 Of note, the deleterious effects of mechanical ventilation in noninjured lungs are only partly dependent on its duration.46 However, an experimental study demonstrated that large VT had only minor (if any) deleterious effects on the lungs, despite prolonged mechanical ventilation.13 Possibly, this finding is explained by the lack of a previous inflammatory insult, as surgery, for example. In fact, it is the systemic inflammation that may prime the lungs to injury by mechanical ventilation.47 OLV with high VT has been shown to induce lung overdistension (volutrauma), promoting VILI.48,49 In patients undergoing abdominal surgery, an intraoperative ventilation strategy with low VT and PEEP improved postoperative lung function50 and even outcome.51 OLV with a VT of 10 mL/kg pbw compared with 5 mL/kg pbw resulted in a higher inflammatory response in a small randomized control trial (RCT).52 In another RCT, OLV with a VT of 6 mL/kg pbw as compared with 10 mL/kg pbw resulted in a lower incidence of postoperative lung dysfunction.5 A metaanalysis of randomized trials on low versus high VT during OLV in patients undergoing thoracic surgery showed a lower incidence of pulmonary infiltrations and ARDS, but the incidence of PPCs did not differ between both strategies.53 A retrospective analysis suggested that the use of low protective VT during OLV may be not sufficient to improve clinical outcomes if not accompanied by appropriate levels of PEEP. In the thoracic surgical patients, Blank et al. analyzed the data (medical records and the Society of Thoracic Surgery database) for postthoracic procedure complications of 1019 patients. Associations between ventilator parameters and clinical outcomes were examined by multivariate linear regression. They found that a Vt of 8 to 9 mL/kg pbw was associated with fewer PPCs. In the large proportion of the patients, the large VT during OLV was inversely propositional to the incidence of respiratory complications.54 The estimation of pbw is crucial when setting the mechanical ventilator for OLV. The use of pbw is based on the assumption that volutrauma might be minimized by delivering a volume appropriate to the patient’s lung capacity.1 Lung capacity and respiratory system compliance are more closely related to height than weight.55–57 pbw for male and female patients can be calculated by the following formulas: It has been suggested that the forced vital capacity (FVC) is a more reliable predictor of appropriate VT for thoracic surgery than pbw,58 and that FVC values below 3.5 L are associated with reduced compliance of the respiratory system. Accordingly, VT equal to functional residual capacity (FRC)/8, as measured preoperatively, could be used to guide the selection of VT during OLV.58 Although adequately powered trials are still missing for a strong recommendation of low protective VT during OLV, values should not exceed 6 mL/kg pbw in most cases.59,60 Low respiratory rates combined with low VT during OLV likely result in hypercapnia. When deliberately conducted, this approach is usually termed permissive hypercapnia, and aims at minimizing VILI.61 Mild hypercapnia can also improve hypercapnic pulmonary vasoconstriction,62 shift the oxyhemoglobin dissociation curve rightward, improve oxygenation,63 thus enhancing oxygen delivery to tissue and, potentially improving wound healing and reducing infectious complications. Besides that, moderate respiratory acidosis resulting in arterial pH of approximately 7.25 can decrease the proinflammatory response during OLV.64 Recently, a comprehensive appraisal of the mechanisms of VILI suggested that the mechanical power transferred from the ventilator to the lung is associated with lung damage.65 Mechanical power represents the energy per unit of time that is delivered to the lungs, and depends directly on the respiratory rate.66 In each breath delivered by the mechanical ventilator, a certain amount of energy is transferred to the patient’s respiratory system, which is mainly used to overcome resistance of the airways and to expand the thorax wall. It has been hypothesized that the extent of lung injury depends on the total amount of “mechanical power” delivered by the ventilator per unit of time. Mechanical power is a single variable which combines volume, pressures, flow, and respiratory rate. Accordingly, lower respiratory rates might contribute to reduce the mechanical power and minimize VILI during OLV. Especially in patients with obstructive lung diseases who show expiratory flow limitation, low respiratory rates should be preferred, to avoid formation of auto-PEEP.67 Because respiratory acidosis may have also undesirable side effects, including increased intracranial pressure, pulmonary hypertension, myocardial depression, and decreased renal perfusion,68–70 its use must be considered judiciously. During OLV, especially in the lateral decubitus position, the dependent lung can by compressed by the mediastinal and intraabdominal structures, as well as surgical manipulation, resulting in further reduction of FRC. PEEP has the potential to keep lung units open at the end of expiration preventing the formation of atelectasis and thereby increasing FRC above the closing capacity of the lungs. Also, appropriate levels of PEEP may result in ventilation within a linear part of the pressure-volume curve of the respiratory system, minimizing atelectrauma.40 However, PEEP can lead also to hyperinflation, increasing the alveolar dead space, and compressing lung capillaries. This might result in an increased afterload of the right ventricle with cardiovascular depression, need for fluid loading, and inotropic support. Furthermore, PEEP in the ventilated lung can shift perfusion to the nonventilated lung, resulting in an increased intrapulmonary shunt. Special caution is needed when setting PEEP in patients with severe obstructive disease, because inspiratory flow limitation may result in dynamic hyperinflation because of auto-PEEP. The appropriate level of PEEP has been a matter of intensive debate in the last decade, and higher levels of PEEP are frequently used. However, recent clinical trials failed to show that high PEEP could improve outcome. In nonobese patients, as well in obese patients submitted to abdominal surgery, a PEEP of 12 cmH2O did not reduce the incidence of PPCs.71,72 Besides using a fixed value, PEEP can be individualized according to different characteristics: (1) the best compliance by a decremental PEEP titration to reach the best compliance;73 (2) the lowest driving pressure;74 and (3) according to electrical impedance tomography (EIT).75 The titrated PEEP may vary depending on the titration method used. PEEP titrated according to the compliance of the respiratory system in patients submitted to abdominal surgery did not reduce the occurrence of PPCs when compared with low PEEP.76 In a small RCT, PEEP level titrated to the lowest driving pressure during OLV, as compared with a fixed level of PEEP of 5 cmH2O, resulted in improved lung protection.74 PEEP values determined with EIT effectively improved oxygenation and lung mechanics during OLV in elderly patients undergoing thoracoscopic surgery.75 However, adequately powered studies on the effects of different PEEP levels on lung protection are missing. The effects of a particular level of PEEP may vary when PEEP is used isolated or in combination with an RM.77 Currently, high-quality evidence is lacking to recommend a routine RM for all patients and further research is required.3 An RM is a maneuver aimed at increasing the transpulmonary pressure in an attempt to revert alveolar collapse.78 Different RMs have been described to achieve this aim in the ventilated, and also the nonventilated, lung. All RMs lead to a temporary increase of the airway pressure, usually with values of 40 cmH2O or more, during 20 to 40 seconds. Most frequently, this is achieved by switching the mechanical ventilator to manual ventilation and using bag squeezing along with appropriate fresh gas flow and setting of the adjustable pressure-limiting valve, as shown schematically in Fig. 20.3. Albeit popular, this procedure is not standardized and may be less effective because loss of airway pressure may occur when resuming mechanical ventilation. The preferred approach is to conduct an RM under pressure-controlled ventilation (PCV), as shown illustratively in Fig. 20.3. If PEEP titration is necessary, changing for volume-controlled ventilation (VCV), followed by decremental PEEP steps of 2 to 3 cmH2O to identify the value leading to maximal compliance of the respiratory system is likely adequate. Alternatively, the RM can be conducted under VCV by increasing VT in increments of 4 mL/kg pbw under a constant high PEEP level of 12 cmH2O. RMs that are conducted under tidal ventilation are usually known as “stepwise RM.” Importantly, during the stepwise RMs, the respiratory rate is reduced to 10/min at an inspiratory to expiratory ratio (I:E) of 1:1, and 1 minute is spent at each VT or PEEP step to allow enough time for lung recruitment during inspiration (3 seconds).
Management of One-Lung Ventilation: Protective Lung Ventilation
Abstract
Keywords
Introduction
Ventilator-Induced Lung Injury
Mechanical ventilation mode
Pressure- or volume-controlled mode
Tidal volume (VT)
≤6 mL/kg predicted body weight (pbw)
Respiratory rate
≤20/min and titrated to arterial pH ≥ 7.25, provided there are no contraindications for permissive hypercapnia
Positive end-expiratory pressure (PEEP)
5–10 cm H2O in most patients, 3–12 cm H2O range possible depending on the other ventilator settings
Driving pressure
≤14 cm H2O
Plateau pressure
≤30 cm H2O
Inspiratory to expiratory ratio (I:E)
1:1 to 1:2
Fraction of inspired oxygen (FiO2)
When switching from TLV to OLV = 1.0
Recruitment maneuvers of the ventilated lung
Not indicated routinely
Protective One-Lung Ventilation
Tidal Volume
Respiratory Rate
Positive End-Expiratory Pressure and Recruitment Maneuvers
Full access? Get Clinical Tree
Management of One-Lung Ventilation: Protective Lung Ventilation
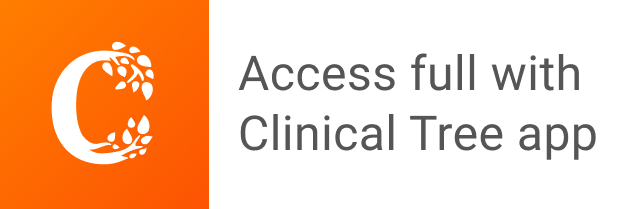