Cardiovascular
Neuromuscular
Electrolyte disturbance
Other
ECG changes
Muscle weakness and fatigue
Hypokalaemia
Nausea and vomiting
Cardiac arrhythmias
Hyperreflexia
Hypocalcaemia
Migraine
Heightened digitalis toxicity
Tremors
Anorexia
Positive Chvostek’s and Trousseau’s signs
Seizures and coma
Signs are not seen until serum Mg2+ falls below 0.5 mmol/L, but this is very variable [4]. Hypomagnesaemia may also cause complications including lactic acidosis [6] and electrolyte imbalances, particularly hypocalcaemia and hypokalaemia due to altered parathyroid hormone (PTH) handling and urinary wasting, respectively [7–9].
Hypomagnesaemia in critically ill patients has been linked to increased morbidity and a decrease in survival time prior to death, with worse averages on prognostic scoring systems such as the Acute Physiology and Chronic Health Evaluation (APACHE) II [10]. Considering the risks associated with low magnesium and the lack of specific signs and symptoms, it is important to maintain a high level of suspicion for low magnesium, particularly in susceptible individuals such as those with history of alcohol abuse, diabetes and chronic diarrhoea [6, 9]. Hypermagnesaemia has a far lower prevalence amongst patient populations. The pathophysiology is commonly iatrogenic, so will be examined in Sect. 13.7.
13.2 Normal Physiology of Magnesium
Magnesium homeostasis is achieved via renal excretion, gastrointestinal absorption and bone resorption. Total magnesium body contents are approximately 22–26 mg [4], which is distributed between bone (53 %), muscle (27 %), soft tissue (19 %) and blood (0.3 %) [5]. Second only to potassium as the cation with highest intracellular concentration of 14–20 mM [3], magnesium has several important cellular roles and is a cofactor for over 300 enzymes, including ATPases and adenylate cyclase. A variety of different mechanisms are employed to achieve this function, such as substrate binding, initiating conformational changes, complex aggregation and binding directly to the active site of an enzyme. This gives magnesium an important role in protein synthesis, metabolic reactions and in the cell cycle. It is functionally vital for the cell, providing stability for nucleic acids, proteins and cell membranes [3, 7, 11].
An example of magnesium’s physiological importance is its role in mitochondrial membrane stability; magnesium protects mitochondria from formation of the permeability transition pore, as illustrated in Fig. 13.1.


Fig. 13.1
The mitochondrial permeability transition pore (MPTP)
The inner mitochondrial membrane (IMM) is a barrier to regulate entry of molecules into the matrix. Oxidative phosphorylation relies on a positive proton gradient and enables formation of ATP.
Increased mitochondrial calcium can trigger pore formation, enabling molecules to pass into the matrix and disrupting oxidative phosphorylation.
Opening of the pore allows any molecule less than 1.5 kDa to cross the normally tightly regulated inner mitochondrial membrane, causing the mitochondria to swell. Subsequently this leads to cellular necrosis due to uncoupling of oxidative phosphorylation in the mitochondrial inner membrane. Alternatively, if the pore subsequently closes, the cell will undergo apoptosis mediated by cytochrome C and other pro-apoptotic molecules, which are released from the damaged outer membrane [12]. Increased levels of mitochondrial calcium can trigger pore formation, which is hindered by elevated extracellular magnesium [12]. One of the proposed mechanisms for this is via inhibition of L-type calcium channels by magnesium, preventing the rise in intracellular and mitochondrial calcium [13].
Magnesium also antagonises calcium in muscle function, neurotransmitter release and cardiac contractility [4, 14]. It has been proposed that magnesium antagonises calcium at the ryanodine receptors of cardiomyocytes by competitively inhibiting calcium activation sites at low calcium ion concentrations and by inhibition at the calcium ion inhibition site at high calcium ion concentrations, resulting in decreased calcium efflux from the sarcoplasmic reticulum [15]. In skeletal muscle, magnesium has been shown to inhibit dihydropyridine receptors to decrease intracellular calcium [15]. The pathway for neurotransmitter release involves phosphorylation of phosphatidylinositol 4,5-bisphosphonate into inositol 1,4,5-triphosphate (IP3), which is subsequently blocked from binding to the IP3-mediated calcium channel by magnesium [5].
13.2.1 Magnesium Absorption and Excretion
Dietary intake of magnesium is estimated to have more than halved in the USA in the last 100 years from approximately 500 to 175–225 mg/day [11] with adolescent females and adult males aged 71 years and over being least likely to have sufficient dietary intake [16]. However, insufficient dietary intake alone is unlikely to result in hypomagnesaemia.
More frequent aetiologies of hypomagnesaemia are increased renal excretion, gastrointestinal losses, decreased gastrointestinal absorption, redistribution or reduced intake with the former two being the most prevalent causes. With regard to normal renal physiology, approximately 80 % total plasma magnesium is filtered through the kidneys of which 3 % is excreted [1, 4, 8, 17]. Reabsorption occurs principally in the cortical segment of the thick ascending limb of the loop of Henle (50–60 %) but the proximal tubule and distal convoluted tubule also have important parts to play, reabsorbing 5–15 and 10 %, respectively [8]. Paracellular magnesium uptake in the cortical thick ascending limb relies on a positive luminal voltage gradient, so the rate of uptake may be altered either by increasing permeability or changing the transepithelial voltage [8]. Negatively charged paracellulin-1 protein has been implicated in this pathway and is expressed in cells in the thick ascending limb of the loop of Henle and the distal convoluted tubule, as shown in Fig. 13.2 [16, 17].


Fig. 13.2
Paracellular pathway
Absorption in the distal convoluted tubule is mediated by transcellular pathways relying on magnesium ion channels MagT1, TRPM6, SLC41A1 and SLC41A2 via as yet unclear mechanisms [7, 8, 16, 17]. Ca2/Mg2 TRPM6 seems to have a significant role, with EGF implicated in the pathway for activation as disabling mutations cause magnesuria [14].
K+ ions are pumped into the lumen in order to maintain a positive transluminal voltage. This, in combination with the negatively charged paracellin 1 molecule, encourages divalent cations such as Mg2+ to be reabsorbed back into the blood.
Gastrointestinal absorption may occur in the entire small intestine and colon [18] but principally magnesium uptake is via the ileum and jejenum [1, 19]. Phytate, fibre and alcohol are several substances that have been shown to reduce magnesium absorption [1, 4, 20]. As with renal absorption, both paracellular and transcellular uptake mechanisms exist, and some studies suggest that although magnesium absorption pathways are distinct from calcium, uptake may be influenced by vitamin D, although this is still debated [4, 17]. Proposed mechanisms for gastrointestinal magnesium absorption include active transport, bulk transport and paracellular uptake [17, 18] but it is not currently understood which mechanisms are predominantly responsibly for magnesium absorption under which conditions.
13.3 Measuring Hypomagnesaemia
It is important to note that patients may be normo-magnesaemic with magnesium deficiency or conversely may be hypomagnesaemic with no physiological magnesium depletion [7, 21]. Measuring serum magnesium has, thus, been debated with regard to its suitability, particularly as a link between serum and intracellular magnesium levels has not been established [4, 21]. Furthermore, there has been much contradictory evidence with regard to the relationship between hypomagnesaemia measured via total serum in critically ill patients and mortality. Some studies conclude that hypomagnesaemia is an effective prognostic indicator [10] while others have found no association [22]. What is certainly not clear is whether the alleged association between hypomagnesaemia and mortality is due to bodily depletion of magnesium.
There are physiological tests available to predict magnesium depletion, such as the magnesium tolerance test. Percentage of retained magnesium is calculated after an infusion, which accurately predicts total body status [4, 7]. Nonetheless, it is reliant upon intact renal excretion, which excludes patients with renal pathologies.
Efforts are being made to find suitable methods for directly measuring ionised magnesium, which is the useful form of magnesium found in the serum. Ionised serum magnesium is usually in the range of 0.54–0.67 mmol/L [4] . However, this has not been easy due to electrode contamination with different cations, particularly calcium. Fluorescent dyes and nuclear magnetic resonance (NMR) spectroscopy are also used in calculating intracellular ionised magnesium but are not routinely available or practical. Currently there are no suitable ways for routinely assessing bodily magnesium status [7, 11, 22].
13.4 Magnesium Deficit: Impact upon the Cardiorespiratory System
There are no pathognomonic ECG abnormalities for hypomagnesaemia but changes seen can include prolonged QT, widened QRS complexes and ST depression. Several cardiac arrhythmias have been linked to hypomagnesaemia of both atrial and ventricular origin, including ventricular tachycardia, atrial tachycardia, atrial fibrillation and torsades de pointes [16].
Indeed magnesium sulphate is a recognised treatment for torsades de pointes irrespective of the patients’ serum magnesium levels [5, 23]. Magnesium’s effect on arrhythmias has been difficult to study due to the commonly seen concurrent hypokalaemia with hypomagnesaemia. One contributing factor for induction of arrhythmias may be that a decrease in intracellular magnesium subsequently decreases potassium, which affects repolarisation during cardiac action potentials [14]. This may be why magnesium deficit can induce digoxin toxicity, although it may also be due to digoxin’s action on the magnesium dependant Na/K-ATPase [5]. At present, however, the exact pathogenesis of arrhythmias in hypomagnesaemia has not been definitively established.
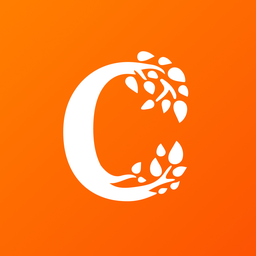
Full access? Get Clinical Tree
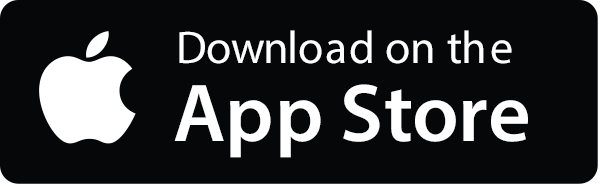
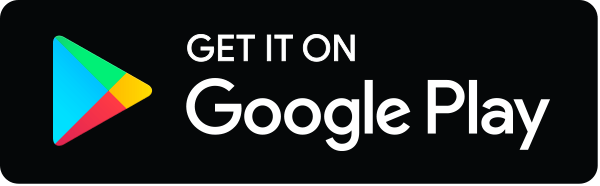